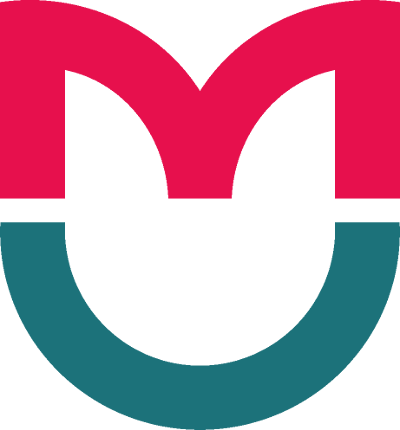
This article is an open access article distributed under the terms and conditions of the Creative Commons Attribution license (CC BY).
METHOD
Combination laser therapy for epiretinal membrane: a physico-mathematical model
1 Pirogov Russian National Research Medical University, Moscow, Russia
2 Research Center for Ophthalmology, Pirogov Russian National Research Medical University, Moscow, Russia
3 Department of Ophthalmology, Faculty of Pediatrics, Pirogov Russian National Research Medical University, Moscow, Russia
4 Stepanov Institute of Physics, Minsk, Belarus
Correspondence should be addressed: Ekaterina P. Tebina
Volokolamskoe shosse 30, bl. 2, 123182; ur.liam@anibetaniretake
Author contribution: Takhchidi KP and Kachalina GF conceived and designed the study; Tebina EP collected and analyzed the data; Kasminina TA performed laser therapy; Zheltov GI, Kasminina TA, and Tebina EP wrote the manuscript; Zheltov GI and Takhchidi KP revised the manuscript.
Ever more people across the world are affected by fibrotic scarring of the retina that can cause significant visual impairment or even blindness [1]. An epiretinal membrane (ERM) is a product of abnormal cell proliferation on the inner surface of the retina and at the vitreomacular interface [2]. It is a fibrous tissue that contracts, causing the retina to pucker and promoting formation of a macular hole [2, 3].
The major contributors to this ocular disorder are posterior vitreous detachment (PVD), the anatomy of the internal limiting membrane (ILM), which has microscopic pores, and abnormal blood supply to the macula [4–6]. According to the literature, ERM is constituted by various cell types, including glial cells (Müller cells, astrocytes and microglial cells), hyalocytes, macrophages, retinal pigment epithelial cells, and fibroblasts [7– 8]. ERM formation is initiated when these cells, regardless of their origin, transdifferentiate into myofibroblast-like cells that abundantly secrete transforming growth factor beta 1, causing the membrane to contract [9–10]. The most intense cell proliferation takes place long before ERM becomes symptomatic. Once the membrane has been formed on the retinal surface, cell proliferation stops [11].
In the early stages of the disease, cell proliferation can be inhibited using a variety of different methods, such as intravitreal injections of high doses of glucocorticoids, radiation therapy or administration of cytostatic agents [12]. These methods, however, have a high risk of adverse effects and therefore are not popular in clinical practice. At present, two strategies are normally used in patients with ERM: surgery to remove the membrane (vitrectomy) and monitoring without treatment [13].
In spite of significant advances in the understanding of the mechanisms underlying ERM formation, the conditions under which vitreoretinal cells transdifferentiate into myofibroblasts are unknown. It is also unclear whether surgical removal of the membrane should be performed in asymptomatic patients or can be safely delayed until visual acuity starts to deteriorate or a patient develops metamorphopsia. Unfortunately, there is no guarantee that the lost vision will be restored after surgery. In addition, post-operative relapses are not a rare thing [12].
Laser therapy is an interesting modality for treating pathologies of the vitreomacular interface [14–15]. The wise choice of laser settings (wavelength, exposure time, power) minimizes damage to the retina and ensures a good therapeutic effect [16–17]. Recently, the authors of this work have patented a new, clinically tested method for treating patients with early stages of preretinal macular fibrosis; the method is a combination of selective photocoagulation of the ERM by a yellow laser and subsequent stimulation of tissue regeneration by a series of subthreshold laser micro-pulses [18]. Such laser therapy induces ERM regression, improves visual acuity and increases light sensitivity of the retina.
Further evolution and clinical application of the proposed method will largely depend on the understanding of its therapeutic mechanisms. Research into biological and physical interactions between the laser beam and structural retinal components can significantly expand our knowledge in this field. a useful tool here is mathematical modeling that accounts for the laser settings needed to achieve a therapeutic effect
[17–20].
We have developed a physico-mathematical model that predicts biophysical effects of laser exposure on retinal tissues. The aim of this study was to investigate and analyze the biophysical response (the dynamics of temperatures and acoustic oscillations, protein denaturation, and stimulation of tissue regeneration) of structural retinal components to a quasi-continuous-wave laser beam and a subsequenta series of laser micropulses.
METHODS
Based on the experiments conducted in animal models (rabbits and primates), we determined the conditions required to induce local changes to intact chorioretinal tissue by laser radiation and identified the underlying mechanisms [17, 19].
These findings, coupled with the knowledge of spectral, optical and physical properties of intraocular structures, laid a basis for developing a physical model of the interactions between the laser beam and ocular fundus tissues (fig. 1) [19]. The mathematical description of this model was used to determine and optimize laser settings needed to preserve the healthy structure of the retina and inhibit cell proliferation in the early stages of ERM.
The model is a multilayer system in which the geometrical dimensions and physical/optical properties of the layers correspond to those of major anatomical structures of the ocular fundus [21]. It accounts for the patterns of laser beam propagation in the anterior part of the eye, light absorption and scattering in the neuroepithelium and the choroid, and some other factors, including the presence of blood flow in the choroid with a speed gradient in the zone extending from Bruch’s membrane to the sclera. Laser beam energy is absorbed by melanin granules and converts into heat. In the model, the optical properties of the intraocular structures located in the anterior part of the eyeball corresponded to those found in individuals above 40 years of age. The concentration of the pigment in the retinal pigment epithelium (RPE) was assumed to be 0.2, which is a typical concentration for a Caucasian patient.
The proposed method for treating ERM uses a combination of grid laser photocoagulation (577 nm wavelength, power of 50 mW, pulse duration of 0.05 s, spot size of 100 μm, distance between the coagulates of 150 μm) and subthreshold laser micropulses (577 nm wavelength, burst length of 30 ms, micropulse duration of 50 μs, duty cycle of 4.7%, repetition rate of 1,000 Hz, spot size of 100 μm, power of 50 mW) [18]. These parameters were used as initial conditions for further computations.
The distribution of temperatures in the chorioretinal complex was determined by a numerical solution of a two-dimensional heat equation [19, 22] fig. 2 shows the equation applied to describe each layer of the model. The size of the irradiated area of the pigment epithelium was assumed to be 100 μm, corresponding to the spot size. Radial irradiance distribution was considered to be Gaussian. The laser wavelength was 577 nm. Given the age of patients and the typical optical loss in the anterior part of the eye, the power of the laser beam absorbed by the chorioretinal complex was assumed to be 0.035 W [21, 23]. Then, given that the beam radius R was 50 μm, irradiance received by the inner limiting membrane was Е ≈ 4.5 • 106 W/m2.
The pulse shape, which defines the Q (t) function, was rectangular.
RESULTS AND DISCUSSION
Below, we describe the temperature profile of the retina irradiated by laser pulses with the parameters specified above. The distribution of temperatures along the laser beam axis (r = 0) is shown in fig. 3. Curve 1 in fig. 3 reflects the degree of tissue heating (T (Z, r = 0)) by the end of the pulse. The averaged value kRPE of yellow light absorption by RPE is 3–10–4m–1. About 30% of energy of the incident light is absorbed by pigment granules of RPE, whereas 70% propagates further into the capillaries and the choroid, and is absorbed by blood hemoglobin, causing tissue heating (Curve 1 in fig. 3).
The part of the choroid adjacent to RPE is heated to the temperatures that exceed that of RPE. This area acts as a buffer, ensuring propagation of heat to the inner layers of the neuroepithelium and preventing the heat flow from traveling deeper into the choroid. Attenuated heat reaches the internal limiting membrane 0.3 s after the onset of exposure. Cooling continues for a few more seconds, stimulating photothermal tissue regeneration in all structural components of the neuroepithelium.
The relatively low absorption value kRPE (in comparison with ki of the green spectrum) means that the degree of a patient’s retinal pigmentation has only a mild effect on the damage threshold. This fact, as well as the lack of absorption of the yellow spectrum by macular pigments, makes the proposed treatment modality especially beneficial for patients [20, 21, 24].
fig. 4 shows the dynamics of tissue cooling and heating T (t, Z, r = 0) in the retinal layers lying in the proximity to the laser beam axis; the colored areas on the graph indicate a dominating optical and thermal effect of laser radiation on the retinal tissues.
Exposure to 0.05-second-long laser pulses results in a temperature rise by about 35 °С and causes denaturation (coagulation) of RPE and proteins of the retina [19, 25]. Under such conditions, about 70% of the protein molecules undergo irreversible damage; therefore, the temperature specified above is considered to be a critical threshold. A temperature rise above the threshold value aggravates damage to tissues. In fig. 3. The area heated above the threshold value is designated as the area of denaturation.
Laser-induced heating of tissue by 10 to 25 °С does not cause irreversible damage, but instead has a therapeutic effect that involves stimulation of retinal tissue regeneration [26]. In the picture, this area is referred to as the area of photothermal stimulation.
Laser-induced heating of tissue by 25 to 35 °С is characterized by a competition between tissue destruction and regeneration. It is difficult to tell which process is dominating; mathematical modelling cannot give an accurate answer. In the figure, this area is termed the staging area.
Summing up, our model predicts denaturation of RPE protein structures and total or partial local coagulation of the retina in the described irradiation modes (Curve 1, fig. 4).
The most pronounced photothermal therapeutic effect on the tissues is observed in the area of inner and outer nuclear layers and part of the inner plexiform layer and ganglia. Here, exposure time is determined by the rate of tissue cooling. It takes the tissues a few seconds to cool down, which is many times longer than the duration of irradiation itself. In the retinal region close to the internal limiting membrane where ERM is formed, the probability of photothermal stimulation remains finite but still decreases considerably (see Curve 5 in fig. 4). This means that laser-induced direct stimulation of ERM growth is highly unlikely. fig. 5 shows 10, 25 and 35 °С isotherms in the r, Z plane; they are given to illustrate radial distribution of temperatures under the studied conditions. The temperature ranges correspond to the data provided in fig. 3.
Thus, the reduction of pulse duration down to 0.03 s shifts the zone of effective photothermal stimulation closer toward the inner nuclear layer. By contrast, an increase in the pulse length up to 0.07 s promotes heating of deeper layers and ERM and, therefore, can directly stimulate its growth.
Thermomechanical effects of laser micropulses on biological tissue
More intricate mathematical modeling is required to predict the thermomechanical effect of short laser pulses on biological tissue [27, 28]. Short pulses induce mechanical (acoustic) oscillations. For yellow laser pulses of 50 μs in length generated at 50 mW, the amplitude of mechanical oscillations approximates decimal fractions of a bar (1 bar ≈ 1 atm). Mechanical (cavitation-induced) damage to biological tissue occurs above the threshold value of 30–40 bar [28, 29].
At power set to 50 mW and a pulse duration of 50 μs, the temperature rise in the hottest spot of the pigment epithelium (along the beam axis) does not exceed 0.15 °С. Given that the repetition rate is 1,000 Hz, the next short pulse arrives in 10–3 s (1 ms). Regardless of the degree of heating, cooling occurs slowly. RPE does not cool down completely in the intervals between the pulses, and, therefore, accumulates heat from pulse to pulse. The interval of 0.03 s is enough to ensure a steady temperature rise of 2 °С. The critical rise above the physiological temperature is 30–35 °С. Generally, the heating and cooling of the retina follow the patterns shown in fig. 2–fig. 4. However, in this case the temperature rise is 20 times lower.
Thus, at the selected stimulation mode, the thermomechanical effect of laser radiation on retinal tissues is very mild. We can almost rule out the probability of inducing thermal damage to the neuroepithelium. The therapeutic effect is determined by the thermomechanical impact of low-intensity laser radiation with the specified parameters.
The efficacy and safety of the proposed method can be illustrated by the following clinical case. Patient I., 68 years of age, presented with complains of decreased visual acuity and distorted vision in the right eye. During the examination, uncorrected visual acuity was 0.6; best-corrected visual acuity was 1.0. Biomicroscopy of the right eye revealed clear optical media. The optic disc was pale-pink, with sharp edges. a “cellophane” membrane was noticed in the macular zone.
The patient underwent multispectral imaging of the retina in the Multicolor mode with the following monochromatic filters: blue (BR; 488 nm), green (GR; 515 nm) and infrared (IR; 820 nm); spectral optical coherence tomography (SOCT), En Face optical coherence tomography angiography (OCT-A) (Spectralis OCT; Heidelberg Engineering, Inc., Germany) and microperimetry (MAIA, CenterVue; Italy). Multispectral imaging revealed the yellow-green foci indicative of ERM prominence (fig. 6A). Measured by microperimetry, the average light sensitivity of the central retina was 26,3 dB (fig. 6B). An OCT scan through the fovea discovered a hyperreflective line intimately associated with the internal limiting membrane; the foveal pit was flat. The thickness of the central retina was increased (257 μm). The outer limiting membrane, the junction between the inner and outer photoreceptor segments, the retinal pigment epithelium, and the choroid were intact (fig. 6C).
Based on those findings, the patient was diagnosed with stage 0–1 preretinal macular fibrosis of the right eye. The patient was offered to undergo laser therapy: a combination of grid laser photocoagulation at 577 nm wavelength and subthreshold laser micropulses at 577 nm wavelength (3 sessions, separated by a month).
The first stage of treatment included grid laser photocoagulation of the retina excluding the avascular zone. The following laser settings were applied: wavelength of 577 nm, power of 50 mW, pulse duration of 0.05 s, spot size of 100 μm, distance between the coagulated spots of 150 μm. Two weeks after the session, a follow-up examination was conducted; uncorrected visual acuity improved to 0.7, best-corrected visual acuity was 1.0.
Multispectral imaging in the Multicolor mode revealed thinner, blurred ERM contours along its entire length, apart from the avascular zones, where coagulation spots remained (fig. 7A). Measured by microperimetry, the average light sensitivity of the retina was 26.5 dB (fig. 7B). SOCT visualized the flat fovea and the same hyperreflective line fused to the retinal surface (ERM). The thickness of the central retina had decreased to 253 μm. The outer limiting membrane, the junction between the inner and outer photoreceptor segments, the choroid, and the retinal pigment epithelium were intact (fig. 7C).
Six months after completing the second part of the treatment course that included 3 sessions of subthreshold laser micropulses (with a month interval between each two sessions), the patient underwent a follow-up examination; uncorrected visual acuity became 0.9, whereas best-corrected acuity was 1.0.
Multispectral imaging conducted in the Multicolor mode revealed regression of the central part of ERM (fig. 8A). Measured by microperimetry, the average light sensitivity of the retina 26.6 dB (fig. 8B). SOCT images revealed formation of the foveal pit; the thickness of the central retina had decreased to 246 μm. The outer limiting membrane, the junction between the inner and outer photoreceptor segments, the choroid, and the retinal pigment epithelium were intact (fig. 8C). Post-operative SOCT images demonstrated that coagulation sites corresponded to the sites of photothermal stimulation showed in fig. 3 and fig. 4.
CONCLUSIONS
We have developed a new laser combination therapy for treating the early stages of ERM. The first clinical tests have confirmed its efficacy and safety. Mathematical modeling allowed us to study the dynamics of thermomechanical effects of laser radiation on the structural components of the neuroepithelium during the exposure. We have also described the major mechanisms underlying the therapeutic effect of laser radiation in patients with ERM.