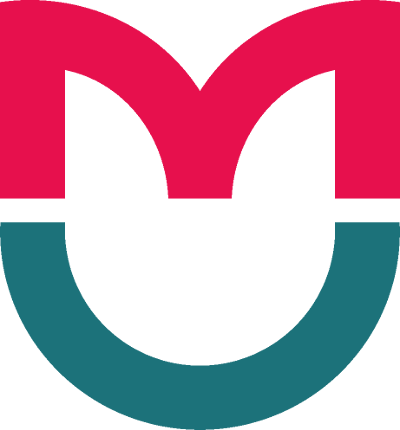
This article is an open access article distributed under the terms and conditions of the Creative Commons Attribution license (CC BY).
ORIGINAL RESEARCH
Effect of robot-assisted gait training on biomechanics of ankle joint in patients with post-stroke hemiparesis
Research Center of Neurology, Moscow, Russia
Correspondence should be addressed: Anton S. Klochkov
Volokolamskoe shosse, 80, Moscow, 125367; ur.ygoloruen@vokhcolk
Funding: this study was state-funded.
Compliance with ethical standards: the study was approved by the Ethics Committee of the Research Center of Neurology (Protocol № 14/09 dated December 23, 2009). Informed consent was obtained from all study participants.
Author contribution: Klochkov AS — study planning, patient recruitment, literature analysis, data interpretation, manuscript preparation; Zimin AA — statistical analysis, data interpretation, manuscript preparation; Khizhnikova AE — literature analysis, data interpretation, manuscript preparation; Suponeva NA, Piradov MA — manuscript preparation.
Gait disturbances are among the most debilitating sequelae of stroke [1, 2]. According to a study, over 30% of stroke survivors were totally unable or required assistance to walk after completing a standard rehabilitation program which did not include robot-assisted gait training [3]. Recent research has shown that exercising with electromechanical gait trainers significantly increases the chance of regaining independent walking [4]. Many authors hold the opinion that the altered biomechanics of the ankle joint are the key factor in gait disturbances, causing the pelvis, the trunk and the hip joint to move in an abnormal, energy-inefficient pattern, which negatively affects the quality and speed of walking and results in compensatory synergies [5–8]. Gait rehabilitation is a stepwise process; compensatory strategies used by the patient at the early stages of rehabilitation are perpetuated over time, becoming pathological due to weakness and spasticity in some muscle groups [9].
Currently, robot-assisted gait training is recognized as the gold standard of locomotor poststroke rehabilitation. Meta-analyses confirm the efficacy of electromechanical devices in restoring the impaired walking function, especially in patients who are unable to ambulate independently [4, 10]. Today, two major types of robotic devices are used in neurorehabilitation: exoskeletons and end-effectors, in which a patient’s feet are fixed to footplates that simulate walking. Exoskeletons can be divided into 2 categories: mobile and stationary. The list of mobile exoskeletons includes but is not limited to ExoAtlet, HAL and Bionic leg, which have proved to be effective in clinical rehabilitation [11, 12]. Our literature analysis did not include publications on mobile exoskeletons because the gait pattern simulated by this technology differs from physiological gait; mobile exoskeletons exploit the zero-moment point concept, i.e. a locomotion model in which the net force is directed towards the surface to ensure safe locomotion; consequently, the ankle joint torque is limited [13–16]. Despite the growing variety of mobile exoskeletons, stationary exoskeletons like Lokomat and ReoAmbulator are more common in clinical practice. So far, both exoskeletons and end-effectors have proved to be clinically effective in helping stroke patients regain their walking function; there is no convincing evidence that one is more beneficial for such patients than the other [17, 18]. Research shows that end-effector-based training increases ankle joint power and kinetics, expands the range of motion of the knee joint, and improves mobility, walking speed and gait symmetry [19, 20]. In turn, stationary exoskeletons ensure precise control over the biomechanics of the assisted movement, reproducing the natural motor pattern and thus allowing the patient to correct the pathological gait pattern [21].
There is ongoing debate about the mechanism underlying the effect of robotic devices for the active training of the hip and knee joints on the changes in the biomechanics of the ankle joint, which is not normally actively involved in the training process. Early works looking into the effect of feedback-enhanced robot-assisted training with an exoskeleton on the biomechanics of voluntary movements during walking demonstrated that the natural kinematic patterns of the hip and knee joints were almost identical to the kinematics prescribed by the robot, whereas torque patterns in the joint remained abnormal, indirectly suggesting that the gait pattern prescribed by the robot could not replace the pattern developed by the patient [22].
The need to involve all joints, including the ankle joint, into training and the hypotheses about the beneficial effects of robotic therapy on the proximal/distal leg joints are a common subject of debate. At present, there are a few commercial robotic exoskeletons with ankle joint drives available on the market. However, they are less popular than exoskeletons with hip and knee joints actuators and have some drawbacks. On the whole, there is no unanimous opinion on whether to equip a gait trainer with an ankle joint actuator and on whether it is enough to stimulate only the proximal paretic leg in order to “overwrite” the pathological gait pattern.
At the same time, it is known that locomotor movements are highly automatized and synergistic. So, it would be logical to hypothesize that gait training that provides active robotic assistance and performance feedback for only some movements generated by muscle synergy also produces an indirect effect on the components that do not participate in performing the trained movement.
The aim of our study was to investigate the possibility of improving ankle joint movements by means of gait training with prescribed knee and hip joints patterns in patients with poststroke paresis.
METHODS
The study conducted in 2010–2017 recruited 22 hemispheric stroke survivors (18 men and 4 women). The median age was 50.5 years (41; 56.5), the median time elapsed after stroke was 6.0 months (2.8; 12.9). Details are provided in tab. 1.
The following inclusion criteria were applied: first-time hemispheric stroke, hemiparesis; gait disturbances.
Exclusion criteria (contraindications to training with the Lokomat) were as follows: weight under 50 kg or over 135 kg; height below 160 cm or above 185 cm; lower limb contractures; persistent skin lesions of lower limbs and the trunk; orthostatic hypotension; severe cardiac pathology; severe cognitive impairment; mechanical ventilation; comorbidities and disorders of the locomotory system, including leg length discrepancy > 3 cm; deep vein thrombosis of lower limbs; hip, knee or ankle arthrodesis; osteoporosis; ununited fractures of lower limbs; prescribed bedrest.
The patients were offered a series of robot-assisted gait training sessions with partial body weight support on the Lokomat (Hocoma; Switzerland) equipped with electromechanical drives in the hip and knee joints for flexion and extension and elastic foot lifters for toe clearance support. A total of 11 sessions were conducted; exoskeleton setup and adjustment took 15 min of the first session; the rest of the session (30 min) was spent on reduced-intensity gait training to allow each patient to adapt to the prescribed gait pattern. The remaining 10 sessions were 45 min long and consisted of only active gait training with sensory feedback. All sessions were one-on-one personal Lokomat trainings sessions conducted by a therapist, with comfortable walking speed and biofeedback based on the hip and knee potentiometers data. Body weight support was adjusted during each session at the minimal amount of support in order to prevent patients from stumbling. Foot support was ensured by tension springs attached distally to the metatarsal. The degree of spring tension was set up by the therapist during each session to assist the patient’s voluntary effort during dorsiflexion and to ensure safe foot clearance.
For performance feedback, the patients were shown graphs illustrating the level of activity and synchronization of their hip and knee joints with the movements of the exoskeleton. The patients were tasked to perform active movements in the hip and knee joints in synch with the exoskeleton. The graphs reflected active movements of the patient; in the absence of active or synchronized movements, the curve dipped. Apart from robotic therapy, all patients received massages for paretic limbs and ten 30-minute long physical therapy sessions to regain their arm function, balance and walking. Within one hour before and after each Lokomat training session, no other therapeutic procedures were carried out.
The clinical efficacy of robot-assisted rehabilitation was assessed using the validated Russian versions of the Fugl- Meyer Assessment (FMA) scale, the modified Ashworth (MAS) scale for spasticity in the gastrocnemius and soleus muscles, the modified Rankin (MR) scale, and the Functional Ambulation Categories (FAC) scale [23, 24]. Measurements were performed before the beginning of the rehabilitation program and on the day following its completion.
The biomechanics of walking were evaluated using the motion analysis system (Biosoft-3D; Russia) [25, 26].
For motion capture, we used reflective markers and 2 synchronized infrared digital cameras. The patients were video-recorded for 30 seconds during a 2-minute walking trial on the treadmill at a comfortable speed before and after rehabilitation. The reflective markers were 2 cm in diameter; they were attached to the anatomic landmarks (projections of joint centers) on the lateral side of the body, including the coracoid process, the most prominent part of the greater trochanter, above the tip of the lateral malleolus, at the heel, and the distal aspect of the foot at the site of the fifth metatarsal head. The kinematic parameters of locomotion were calculated in the XYZ coordinates (Z — vertical axis; Y — sagittal axis, running from right to left; X — frontal axis). The following kinematic parameters were calculated:
– marker coordinates on the X, Y, Z axes and their movement trajectories;
– angles between body segments and the X, Y, Z axes;
– joint angles.
The second-order low-pass Butterworth filter was applied to the obtained raw data. Kinematic parameters were calculated based on the 3-D coordinates of the reflective markers and the constructed kinetogram of the human body. Temporal characteristics of gait (duration of the stance and swing phases) were used as basic parameters for primary gait analysis; they were calculated based on the local vertical acceleration maximum for the markers attached to the lateral malleolus and the heel plus the local horizontal acceleration maximum for the markers attached to the fifth metatarsal head. Other primary kinematic parameters included maximums and ranges of torque and joint angles in the sagittal plane of the ankle joint. For secondary data analysis, cluster analysis and nonparametric analysis of ten- percentile intervals were used.
Cluster analysis is widely used to study gait in general and the gait cycle in particular in healthy and diseased individuals [27–35]. In this work, cluster analysis was applied to identify the structure of the gait cycle and to compare its characteristics before and after rehabilitation. Nonparametric analysis of ten-percentile intervals of the gait cycle was conducted to study the characteristics of the cycle in greater detail and compare them before and after gait rehabilitation.
The obtained data were processed using nonparametric tests: the Mann–Whitney U test for independent variables and the Wilcoxon test for dependent variables. The Bonferroni correction was used to adjust for multiple comparisons. Categorical variables were analyzed using the Fisher exact test. To identify the hierarchy of the obtained data, cluster analysis was applied. Specifically, Ward’s method followed by the Mann–Whitney test and the Bonferroni correction procedure was used to identify the components of the gait cycle and subgroups of patients. The strength of associations was evaluated using Spearman’s correlation coefficient. Results are presented below as median values, upper and lower quartiles. Differences were considered significant at р < 0.05. The analysis was carried out in Statistica v. 7.0 (StatSoft Inc; Russia) and SPSS 22 software (IBM; USA).
RESULTS
Clinical efficacy of gait training
The analysis of clinical data showed that patients’ mobility had improved following the rehabilitation program; another finding was an insignificant yet reliable increase in the range of active motion and function of the lower limb and a reduction in gastrocnemius and soleus spasticity in all the participants; the initial degree of disability on the Rankin scale remained unchanged. After gait rehabilitation, a higher proportion of patients could ambulate without assistance than before they joined the program (3, 4 and 5 points on the FAC scale; fig. 1).
We also observed an increase in active motion on the FM scale after rehabilitation. The total motor score increased from 146.5 (128; 163.5) to 152 (134.3; 176.8) (p < 0.05), whereas for the lower extremity, the score increased from 18 (16; 21) to 20.5 (18; 24.3) (p < 0.05).
The tone of the gastrocnemius and soleus muscles decreased significantly from 2.5 (1; 3) to 2.0 (0; 2) points on the MAS (p < 0.05). Details on the distribution of muscle tone parameters are provided in fig. 2.
Assessment of gait biomechanics
Temporal and spatial characteristics of gait changed following robot-assisted gait rehabilitation: the duration of the stance phase increased from 28.0% (25; 36) to 33.5% (30; 42) relative to the gait cycle (GC) (p = 0.0001).
The analysis of kinematic parameters did not reveal any significant differences in the maximum values for ankle joint flexion and extension during GC. Maximum angle ranges before and after rehabilitation were 59.12° and 45.30°, respectively, the difference being insignificant (р = 0.228). Minimum angle ranges were 3.33° and –1.79°, respectively (р = 0.072). The differences between the maximum and minimum values were 55.79° and 47.09°, respectively (р = 0.190). However, comparison of the median values of GC ten-percentile intervals before and after rehabilitation revealed that initially different patients’ goniograms looked more similar after rehabilitation (fig. 3А–C).
Considering the absence of significant differences in the primary kinematic parameters, we conducted an in-depth analysis of goniogram variability and ankle joint torques (tab. 2).
To determine the degree of data variability, we analyzed the correlation coefficients for the angles between each two consecutive GC parts. Based on the graph (fig. 4), we concluded that differences between the patients were the most pronounced at 51%–58% and 65%–82% of the gait cycle.
For a more in-depth analysis of ankle joint goniograms constructed before and after rehabilitation, we broke down the gait cycle into parts using cluster analysis and correlation coefficients for each 2 consecutive angles. Using Ward’s clustering technique, the gait cycle before rehabilitation was divided into 5 parts corresponding to 1–7%, 8–19%, 20–55%, 56–74%, and 75–100% of GC. The first part corresponded to the time of foot contact with the treadmill and the beginning of the stance phase; the second part corresponded to the middle of the stance phase; the third part, to the end of the stance phase and the beginning of the swing phase; the fourth part, to the middle of the swing phase; the fifth part, to the end of the swing phase. Cluster analysis was applied to each GC part. Descriptive statistics for GC parts before clustering are provided in tab. 3.
After 5 parts were identified in the pre-rehabilitation gait cycle, the patients were clustered into subgroups for each of those parts. Two clusters were identified in the first part of the cycle (1–7%). The second part (8–19%) was represented by 3 clusters, differing in their medians. In the third (20–55%) and fourth (56–74%) parts, 3 and 4 clusters were identified, respectively, differing in medians. The fifth part (75–97%) was represented by 3 clusters. Based on the post-rehabilitation goniograms, Ward’s clustering identified 3 parts in the post-rehabilitation gait cycle at 1–61%, 62–75% and 76–97% of the cycle. There were 3 clusters identified in the first part, 2 clusters in the second, and 3 clusters in the third. Thus, the main difference in the GC structure before and after rehabilitation was the presence of 3 parts instead of 5, suggesting consolidation of the goniograms at 1–61% of the gait cycle. Angle comparison before and after rehabilitation demonstrated significant differences in IR only (р < 0.05).
The analysis of torque medians in the ten-percentile intervals of the gait cycle before and after rehabilitation also revealed consolidation of these parameters after completion of the rehabilitation program; the most pronounced differences in torques were observed during dorsiflexion in the swing phase (fig. 5А–C).
Comparison of torque medians in the ten-percentile GC intervals for each patient before and after rehabilitation revealed that torque patterns became more similar after rehabilitation. In almost all our patients, torque peaked during the 7th ten-percentile interval of the gait cycle (71–80%), which corresponds to the middle of the swing phase. The second, not so pronounced peak was observed in the 10th ten-percentile interval of the cycle (91–97%), which corresponds to the end of the swing phase. Statistical analysis showed that the observed changes were associated with Q3 changes and joint torque peaks. During the second half of pre-rehabilitation GC, 3 torque peaks were detected at 55%, 75% (the absolute maximum) and 95% of the cycle. After rehabilitation, there were also 3 torque peaks; however, the first and second peaks almost “fused” into a single peak. After rehabilitation, the first and the second torque peaks were lower than before the program; the third peak was almost the same before and after rehabilitation (fig. 6).
Summing up, there was an increase in voluntary activity of the lower limb on the FMA scale, a reduction in gastrocnemius and soleus muscles spasticity on the MAS and functional improvement on the FAC scale in patients with post-stroke hemiparesis after robot-assisted gait rehabilitation.
Thorough analysis of movement biomechanics allowed us to identify changes in the GC structure (an increase in the duration of the stance phase) and ankle joint angles/torques (a reduction in the variability and fusion of torque peaks).
DISCUSSION
GC asymmetry is one of the most common gait disturbances beside reduced walking speed and shorter step length observed in stroke survivors. In GC asymmetry, the stance phase becomes shorter, whereas the swing phase of the paretic limb becomes longer, which is reflected in the temporal characteristics of the gait cycle before rehabilitation [2]. The increase in the duration of the stance phase from 28.0% (25; 36) to 33.5% (30; 42) relative to the entire length of the GC following gait training might be the result of improved strength in the muscles supporting the paretic limb, improved weight shifting, reduced spasticity, and better coordination between the joints. It is known that high muscle tone of ankle flexors prevents the foot from dorsiflexion, forcing the body to stop its forward propulsion; the center of gravity is thus left behind the ankle joint line, the swing phase of the healthy leg becomes shorter, resulting in a shorter step. According to some authors [36], robot-assisted training can reduce spasticity in ankle extensor muscles and indirectly affect the recovery of gait symmetry. This hypothesis is supported by our findings concerning muscle tone reduction in the gastrocnemius and soleus muscles. Reduced muscle tone might promote ankle dorsiflexion during the stance phase. However, no significant differences in the range of motion during the stance phase were detected, which might be explained by the limited amplitude of the ankle dorsiflexion movement in the middle of the stance phase (the range of motion does not exceed 10° at the moment when the center of mass passes the center of pressure) [37]. At the same time, consolidation of ankle joint goniograms before the time point corresponding to 61% of the gait cycle (this part includes the stance phase and the beginning of the swing phase) indirectly suggests a positive effect of the prescribed physiological pattern of the center of mass movement on the ankle joint kinematics during the stance phase.
Apart from the stance and swing phases, some researchers identify 6 phases in the physiological gait biomechanics designated by the peak values of its dynamic parameters [38, 39]. Among these phases, the part at 60–73% of the GC corresponds to the acceleration phase, which begins when the foot leaves the ground and ends when the swing leg is adjacent to the stance leg. In this phase, the body gains up speed to advance the leg forward. In the next part of the cycle (73–87%) the foot passively advances further. This phase begins when the swing leg is adjacent to the stance leg and ends when the swing leg is in front of the body and its tibia is vertical [2, 40].
We found that in patients with poststroke paresis the biomechanics of the ankle joint were changed after gait rehabilitation: the joint was stable during the stance phase and the dorsiflexion movement was faster and smoother as the leg was advancing forward, ensuring sufficient foot clearance. Such changes might be promoted by the synergy of the hip and knee joints prescribed by the robotic trainer and the lack of opportunity to use compensatory strategies for better foot clearance. Perhaps, robot-assisted gait training has a certain universal tuning effect on the ankle joint movement resulting from the correction of the compensatory synergy of the hip and knee joints, reflected in the reduced data variability (р < 0.05; Mann–Whitney U), smaller number of the identified clusters and less differing medians after rehabilitation. However, patient clusters identified before and after rehabilitation were very different. This leads us to hypothesize that since patients move to other clusters as they continue gait training, the effect is achieved through different mechanisms, despite the overall “leveling” effect of rehabilitation on the gait pattern.
Comparison of the total sum of angles in the gait cycle reveals that peak values leveled out as other patients showed a shift towards the median. Therefore, we conclude that there were no significant changes in the total sum of ankle joint angles after rehabilitation (р = 0.521; Mann–Whitney U). This suggests that structural changes in the gait pattern are not determined by quantitative parameters but are largely the result of the shift in the gait pattern towards the values characteristics of the average gait pattern. Some studies demonstrate that ankle joint angles change following gait rehabilitation, leading to improved dorsiflexion, but those changes were detected in patients with moderate motor deficit in the ankle joint [40].
Hypothetically, therapy can target not the compensatory muscle synergy as a whole, but some of its components. If its components are more or less equally important, it does not matter which one is directly targeted. However, if the compensatory muscle synergy is not completely formed, the best correctional effect can be achieved by targeting the movement that initiated the development of compensatory synergies.
Our study has a few limitations. During motion capture, the patients were walking on the treadmill which was not equipped with force plates, so we were unable to analyze support reaction forces. To better understand the biomechanics of the studied joints, further research should be focused on comparing patients and healthy individuals and analyzing the follow-up data and EMG results for muscle activity.
CONCLUSIONS
Robot-assisted gait training of the hip and knee joints can cause changes in ankle goniograms and ankle joint torques in patients with pathological synergies, improving the biomechanics of the ankle joint. Further research should focus on the comprehensive analysis of the movements of the paretic and healthy leg, pelvis and trunk. Besides, in order to understand the mechanisms promoting changes in muscle synergies, the study protocol should include groups with different exposure to training. The analysis of the effect that robotic therapy has on complex movements will allow to design new training protocols accounting for the presence and severity of pathological compensatory gait patterns.