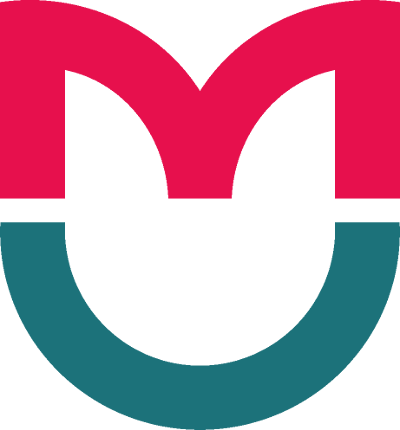
This article is an open access article distributed under the terms and conditions of the Creative Commons Attribution license (CC BY).
METHOD
Tissue chemiluminescence as a method of evaluation of superoxide radical producing ability of mitochondria
Department of Medical Biophysics, Faculty of Fundamental Medicine,Lomonosov Moscow State University, Moscow, Russia
Correspondence should be addressed: Aishat Dzhatdoeva
Lomonosovskiy prospekt, d. 31, korp. 5, Moscow, Russia, 119192; moc.liamg@jdtahsya
Funding: this work was supported by the Russian Science Foundation (project no. 14-15-00375).
Acknowledgments: authors thank Aleksey Grishin, a student at the Department of Physiology, Lomonosov Moscow State University, for his help in choosing gas mixture components.
Human mitochondria, apoptosis and diseases
Mitochondria are important organelles found in cells. They are vital because they perform a variety of functions. But above all, they generate energy through oxidative phosphorylation and regulate growth, aging and cell apoptosis. There are about 400 mitochondrial diseases recognized. Also, mitochondrial medicine has developed as an independent scientific direction.
Cellular energy dysfunction may lead to neuromuscular abnormalities [1]. Nerve tissue is particularly sensitive to reduced energy metabolism [2]. However, energy dysfunction may not be the most dangerous mitochondrial dysfunction. Mitochondria are the major source of formation of intracellular free radicals [3], first of all superoxide anion radical (SAR). Accumulation of SAR and its derivatives — reactive oxygen species – leads to mitochondrial oxidative stress. This is extremely dangerous for cells because it can trigger programmed cell death called apoptosis [4]. There are two basic pathways to triggering apoptosis: external (receptor) and internal (mitochondrial) pathways [5]. Most forms of apoptosis in vertebrates come through the second pathway [6].
Oxidative stress in mitochondria is known to be the causative factor or pathogenesis link of many diseases: neurodegenerative diseases (Parkinson’s disease [7], Alzheimer’s disease [8], multiple sclerosis [9], amyotrophic lateral sclerosis, and others), neuro-ophthalmopathy, glomerulonephritis [10], insulin resistance, as well as aging. Higher risk of a number of diseases (cancer, diabetes [11], cardiovascular diseases) is associated with polymorphisms of antioxidant enzymes, manganese-dependent superoxide dismutase (MnSOD) and glutathione peroxidase, which arrest the consequences of mitochondrial oxidative stress. The essential role of mitochondrial dysfunction in the development of cancer was also identified [5].
Mitochondrial dysfunction and accumulation of free radicals in the cell are influenced by adverse factors. One of such factors is gene mutation. Unlike other organelles, mitochondria have deoxyribonucleic acid (mtDNA), which encodes a subunit of some complexes of oxidative phosphorylation. Mutations in mtDNA, as well as the genes of nuclear DNA that encodes mitochondrial proteins cause Leber’s hereditary optic neuropathy (or Leber optic atrophy) [12], NARP (Neuropathy, Ataxia, and Retinitis Pigmentosa) syndrome [13], MERRF (Myoclonus Epilepsy with Ragged-Red Fibers in skeletal muscle) syndrome, MELAS (Mitochondrial encephalomyopathy, lactic acidosis, and stroke-like episodes) syndrome [14], Kearns-Sayre syndrome (retinitis pigmentosa, external ophthalmoplegia, and complete heart block, ptosis, cerebellar syndrome) [15], Pearson syndrome (abnormal functioning of the bone marrow, liver, and pancreas) [16], and others.
Mitochondria as sources of free radicals in apoptosis
During aerobic respiration, there is leak of 1.2 % of electrons from the mitochondrial electron transport chain, which restores oxygen with production of superoxide anion radical [17]. Complex I and complex III of the respiratory chain are the main centers of production of reactive oxygen species. Normally, the body’s antioxidant system neutralizes free radicals formed. However, under the influence of adverse factors, the level of free radicals increases by many times.
First, electron transfer is blocked in the mitochondria [18] resulting in respiratory depression, reduced synthesis of adenosine triphosphate (ATP) and, most importantly, increased formation of mitochondrial superoxide anion radical [19]. Then, under the influence of mitochondrial superoxide, hydrogen peroxide is formed in the matrix. After that, the complex of mitochondria-specific phospholipid — cardiolipin with cytochrome c is formed [20]. As demonstrated in model experiments, this complex has peroxidase activity [4]. In the presence of hydrogen peroxide, it oxidizes organic substrates, including polyunsaturated fatty acids. This leads to production of lipid free radicals [21] and triggers a chain reaction of lipid peroxidation [22]. Lipid peroxidation in mitochondrial membranes leads to swelling of the matrix [23], rupture of the outer membrane or at least formation of large pores in it through which cytochrome c leaves from the mitochondria. Appearance of cytochrome c in the cytoplasm triggers apoptosis reaction [24].
Methods of assessing the radical-producing ability of mitochondria in a living tissue
Various methods, including chemiluminescence (CL) [25], are used to assess the radical-producing ability of mitochondria in a living tissue. CL allows to register the concentration of radicals (which is extremely low in living systems) and the rate of reaction in which the radicals participate. A method for registering tissue CL using a refrigerated photomultiplier [26] has been recently developed. This method has several advantages over the labor-consuming and costly method by Japanese researchers using a refrigerated photomatrix [27]. The essence of the proposed method for assessing the radical-producing ability of a tissue [26] is that under regulated temperature (37 °C), weak air flow is fed from a peristaltic pump, through a capillary, to a system comprising of the test samples of the tissue and lucigenin activator (fig. 1A). The selected position level of the capillary and the aeration rate allow to observe formation of superoxide anion radicals in tissues affected. However, a study [26] showed CL to rise over time, which complicates analysis of curves obtained. Possibly, additional CL amplification is associated increased pH value of the medium solution over time, and not with additional production of radicals in the tissue. It is known that the intensity of lucigenin luminescence depends on the pH of the medium [28]. Increased pH of the medium enhances production of lucigenin cation, which interacts with SAR, followed by emission of quantum of light.
Our study included two experiments. The first experiment was aimed at determining the gas mixture composition optimal for maintenance of a constant pH level at aeration of prototypes using the method studied. The aim of the second experiment was to assess the level of production of superoxide anion radicals in hypoxia and parkinsonism in the tissue samples of rat brain and mouse heart using the studied method.
METHODS
Installation scheme
SmartLum-l100 chemiluminometer (DISoft, Russia) additionally equipped with peristaltic pump Pumps 323 (Watson Marlow, UK) was used in the experiments. Tanks containing different gas mixtures were connected to the pump. The installation scheme is shown in fig. 1A.
The distance (l) from the bottom of the cuvette to the tip of the capillary supplying gas to the working solution was 1.5 cm (fig. 1B). This contributed to rapid diffusion of gas to the test sample without disturbing its position in space. Slices were placed at the bottom of the cuvette making the sample side with the largest area to face the detector (fig. 1B).
Animals and manipulations
The study used the heart of white male Wistar rats aged 2–3 months and the brain of C57BL/6 mice aged 2.5–3 months. While working with the animals, regulations (No 755, Order of August 12, 1977) established by the Russian Ministry of Health were complied with. The Bioethics Commission of Lomonosov Moscow State University approved the experiments. The animals were kept in a vivarium, 6 animals each in standard T4 cells with controlled lighting (12 hours in the night and 12 hours in the day), with free access to feed and water.
Rats. All organ-harvesting manipulations were performed after the animals were deeply anesthetized with chloral hydrate (400 mg/kg). After harvesting the organs, they were washed in saline solution (0.9 % NaCl). A sharp blade was used to cut out a small rectangular piece of the left ventricle, not more than 5 x 5 x 5 mm in size and then washed.
Mice. To model the early symptomatic stage of Parkinsonism, a 12 mg/kg dose of proneurotoxin 1-methyl-4- phenyl-1,2,3,6-tetrahydropyridine (MPTP; Sigma, USA) was administered subcutaneously to the mice four times, at two- hour intervals between injections [29]. The control animals were administered with a saline solution (0.9 % NaCl) in the same way. 12 hours after administering the last proneurotoxin dose, the mice were decapitated (without anesthesia) and the brain isolated. Thin frontal slices, 300 microns thick, were obtained using vibratome (Vibratome 1000 Plus, USA). Tissue blocks containing the substantia nigra (place of localization of the bodies of dopaminergic neurons) and striatum (area of axonal projections of dopaminergic neurons) were isolated in the slices.
To preserve tissue viability, all manipulations were performed in ice-cold Krebs-Ringer solution, comprising of NaCl 6.96 g, KCl 0.36 g, CaCl2 0.22 g, MgSO4•H2O 0.33 g, NaHCO3 2.1 g, D-glucose 1.82 g, HEPES 4.8 g, distilled water 1.0 l. After that, the pH level was brought to 7.4. The experiments used only a freshly prepared solution.
Determining the optimal composition of the aeration gas mixture
The optimal composition of aeration gas mixture was determined using the heart tissue samples of rats. The tissue sample was placed in a cuvette containing 90 μm of lucigenin in Krebs-Ringer solution. Lucigenin was used as a selective probe for SAR. The chemiluminescence of tissue samples were registered for 125 minutes under controlled temperature (37 °C) and aeration at pump rotor speed of 6 rpm. Such a rate of gas mixture supply allowed to maintain sufficient rate of saturation of the solution with gas, mix the solution and wash the sample, keeping it fixed at the bottom of the cuvette. The pH of the solution was measured every 20 minutes.
Three gas mixtures of the following composition were examined:
- atmospheric air: 21 % O2, 0.03 % CO2, 78 % N2,
- carbogen: 95 % O2, 5 % CO2,
- human exhaled air: 15 % O2, 4 % CO2, 74 % N2, others – 7 %.
Registration of tissue chemiluminescence in hypoxia simulation and in Parkinsonism
Based on the results of the experiment carried out to determine the optimal composition of the aeration gas mixture, technical mixtures of new composition were prepared and were used for chemiluminescence registration in modeling of hypoxia and parkinsonism:
- oxygen-containing gas mixture (OCGM): 15 % O2, 5 % CO2, 80 % N2,
- oxygen-free gas mixture (OFGM): 5 % CO2, 95 % N2.
Hypoxia modeling. The study was conducted on samples of rat heart tissue using three hypoxia models. The CL of one sample was registered for each model. To create hypoxic conditions, anoxic gas mixture was passed through a solution containing a piece of the tissue. To create reoxygenation conditions, oxygen-containing gas mixture was passed. Three hypoxia models were investigated.
Model 1 — hypoxia for 15 minutes. For the first 30 minutes, CL was registered at OCGM aeration, followed by 15-minute CL registration at OFGM (hypoxia) aeration. After that, OCGM aeration was resumed for 30 minutes (reoxygenation). This hypoxia cycle was repeated six times. The total CL registration time was 400 minutes.
Model 2 — hypoxia for 150 minutes. For the first 30 minutes, CL was registered at OCGM aeration, followed by 150-minute CL registration at OFGM (hypoxia) aeration. After that, OCGM aeration was resumed for 30 minutes (reoxygenation). This hypoxia cycle was repeated twice. The total CL registration time was 325 minutes.
Model 3 — hypoxia for 240 minutes. For the first 30 minutes, CL was registered at OCGM aeration, followed by 240-minute CL registration at OFGM (hypoxia) aeration. After that, OCGM aeration was resumed for 30 minutes (reoxygenation). The total CL registration time was 350 minutes.
Change in radical formation was assessed by S/S0 parameter, where S is the area under the CL curve within 30 minutes of reoxygenation at the end of the experiment with the model. Parameter S reflects the amount of radicals formed. S0 is the area under the CL curve over the first 30 minutes of luminescence registration. S0 reflects the initial amount of radicals. Unit of measure S — (imp/s) × min.
Modeling of Parkinsonism. The study was performed on mouse brain slices. The CL of three tissue slices of the area of the substantia nigra and the three sections of the striatum area of the brain (of both experimental and control animals) was registered. Chemiluminescence was registered over 25 minutes under aeration with oxygen-containing gas mixture. SAR formation was assessed by S/S0 parameter (after 20 minutes of aeration), where S is the area under the CL curve of the tissue of the experimental animals, while S0 is the area under the CL curve of the tissue of the control animals.
Data were statistically processed using software packages Statistica 7.0 and MS Office Excel 2010. The results were presented as mean value and standard deviation. The significance of differences between the groups was determined using the Mann–Whitney U test. The differences were acknowledged to be statistically significant at a significance level of p < 0.05.
RESULTS
Dependence of pH on the composition of the aeration gas mixture
The influence of the composition of three different gas mixtures on the pH level of a solution containing a sample of the heart tissue was investigated. With atmospheric air aeration, pH rose from 7.4 to 9.0 (fig. 2A). A change in the pH value influenced the intensity of lucigenin-dependent chemiluminescence. The registered changes in the kinetics of the CL curve are a direct consequence of the alkalinity of the medium: the growth dynamics of CL and increase in pH coincided over time (fig. 2).
On the contrary, aeration with gas mixtures with high content of CO2 (carbogen and exhaled air) did not result in a significant change in pH of the medium during the experiment. CL luminescence remained at a constant level (fig. 2B).
Thus, CO2 content in gaseous mixtures at a 4–5 % level is sufficient to maintain the pH at physiological norm (7.4). Therefore, for further CL registration of tissue samples, technical gas mixture was prepared in which the percentage content of the main components was similar to the exhaled air. However, there were no impurity gases (OCGM). Oxygen-free gas mixture (OFGM) with high content of CO2 was used to create hypoxic conditions.
Formation of free radicals in the heart tissues of rats in hypoxia
Under repeated cycles of hypoxia lasting for 15 minutes, a significant increase in the formation of superoxide anion radical was observed only at the time of reoxygenation at the 300th minute of the experiment: the amount of SAR increased by 1.5 times in comparison with the baseline. Under longer cycles of hypoxia (150 and 240 minutes), statistically significant increase in CL was also observed at the end of the experiment. Here, formation of SAR increased by 1.8 and 2.0 times respectively (fig. 3, tab. 1).
Formation of free radicals in the brain tissues of mice in parkinsonism
The modeled stage of parkinsonism in mice corresponds to early symptomatic stage in people. At this stage, increased formation of superoxide anion radical was observed in the animals. In the case of brain tissue slices containing the striatum, a significant increase (1.7 times) in SAR production was detected. For brain tissue slices containing the substantia nigra, there were no significant differences between the experimental and control animals. However, a tendency towards 1.3 times increase in production of radicals was detected (fig. 4, tab. 2).
DISCUSSION
Lucigenin-enhanced chemiluminescence is a promising method for measuring the level of SAR production and assessing disorders that occur in individual cells and the tissue in general. However, applying his method requires maintenance of a constant pH of the medium at 7.4. When aerating samples with atmospheric air, the pH index changes, while the volume of the Krebs–Ringer buffer system (2 ml per 1 mg tissue) is not enough to stabilize the pH at 7.4. On one hand, the use of a continuous-flow system can help solve the identified problem by constantly renewing the solution used to wash the tissue. However, the necessary technical retrofit measures and increased reagent consumption make this approach difficult to implement. On the other hand, the possibility of changing the composition of the gas mixture for aeration of the solution — increasing the CO2 content — is a simpler and more affordable method. Saturating the washing solution with carbon dioxide allows to maintain the pH at a constant level by dissolving the gas in water and establishing the balance state:
We showed that aeration with a gas mixture containing 4–5 % of carbon dioxide is optimal for the method.
The hypoxia/reoxygenation cycle is known to play a key role in human infarction. Moreover, the tissue suffers the most severe damage at sharp increase in production of reactive oxygen species and dies after resumption in blood supply. Assessment of the level of formation of superoxide radical anion at different periods of hypoxia showed that the cyclical effects of short periods of hypoxia led to lower production of free radicals than longer periods of hypoxia.
Registration of lucigenin-enhanced CL under aeration of tissue sample with oxygen-containing gas mixture showed an increase in formation of SAR in the brain tissue 12 hours after the last dose of proneurotoxin was administered. This suggests that increased production of free radicals, leading to degeneration of nerve cells, occurs long before the first clinical symptoms of the pathology appear.
CONCLUSIONS
Conditions for the use of lucigenin-enhanced chemiluminescence to evaluate the radical-producing ability of biological tissues were optimized. Compositions of oxygen- containing and oxygen-free gas mixtures for aeration of the test sample to maintain at 7.4 the pH of the solution used to wash the sample were selected.
The possibility of using the method for estimation of the level of production of radicals in hypoxia and parkinsonism was demonstrated. There was significant increase in the level of production of radicals in heart tissue for hypoxia cycles — 1.8 times increase for 150-minute hypoxia cycle, and 2.0 times increase for 240-cycle. The level of SAR production in Parkinsonism in areas of the striatum and substantia nigra 12 hours after the last dose of proneurotoxin was administered was 1.7 and 1.3 times higher respectively than in the control sample.