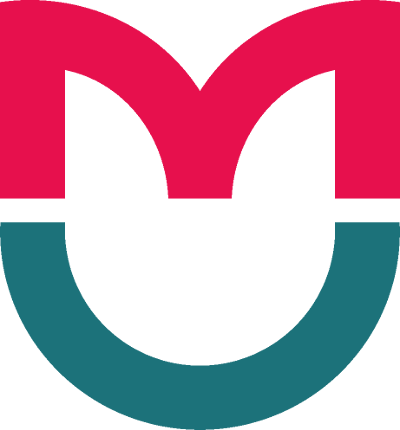
This article is an open access article distributed under the terms and conditions of the Creative Commons Attribution license (CC BY).
ORIGINAL RESEARCH
Telomerized fibroblasts as a candidate 3D in vitro model of pathological hypertrophic scars
Orekhovich Research Institute of Biomedical Chemistry, Moscow, Russia
Correspondence should be addressed: Valerian S. Shadrin
Pogodinskaya, 10, str. 8, Moscow, 119121; moc.liamg@nirdahsnairelav
Funding: this research was supported by the Russian Ministry of Science and Higher Education and was conducted under the Federal Targeted Program on Research and Development in Priority Fields of Science and Technology for 2014–2020 (Agreement 05.604.21.0219, Project ID RFMEFI60419X0219).
Author contribution: Luzgina NG, Rusanov AL conceived the study and proposed its design; Shadrin VS, Kozhin PM, Shoshina OO, Luzgina NG, Rusanov AL analyzed the literature, analyzed and interpreted the experimental data and wrote the manuscript; Shadrin VS, Kozhin PM planned and conducted the experiment; Shadrin VS wrote the manuscript.
Every year, over 100 million people worldwide develop skin scars after surgical interventions, injuries or burns. Wounds heal differently depending on their location, depth, healing conditions, and clinical characteristics of the affected patients; wound repair can culminate in the formation of a normal (normotrophic) or pathological scar. Stimulated by a variety of factors, abnormal fibrous tissue growth leads to hypertrophic and keloid scarring. Both types of scarring are a cutaneous fibroproliferative disorder characterized by the hyperactivation of fibroblasts in the wound and excessive proliferation of extracellular matrix [1].
The absence of simple and cost-effective in vitro cell models replicating profibrogenic pathways makes it difficult to explore the pathogenesis of hypertrophic and keloid scarring and to develop effective preventive and curative treatments. Fibroblasts isolated from keloid tissue vary in phenotype between donors; besides obtaining the sufficient amount of clinical keloid tissue specimens is a real challenge. Therefore, the search for the optimal cell model for reproducing the phenotype of keloid fibroblasts is a pressing concern. It seems promising to use immortalized cell lines that can proliferate indefinitely and retain a stable phenotype at high passage numbers.
It is known that keloid fibroblasts are characterized by high proliferative activity, rapid growth, increased expression of collagen I, fibronectin, elastin, periostin (regulates production of collagen I), and tenascin (participates in cell adhesion) [2, 3], resulting in extensive connective tissue growth beyond the borders of the injury. Some aspects of keloid fibroblast metabolism are similar to those of tumor cells [4, 5].
It is hypothesized that the fibroblast phenotype observed in pathological scars is determined by high telomerase activity [6]. A research team was able to suppress proliferation and growth of keloid fibroblasts and normalize some of their phenotypic traits by decreasing telomerase activity in these cells; the authors concluded that telomerase inhibition might be a promising strategy for keloid therapy [5]. Therefore, it would be interesting to evaluate the potential of telomerized fibroblasts for the in vitro modeling of pathological scarring.
Optimization of cell culture techniques is crucial for designing an adequate scarring model. It is known that the center of keloid tissue is often hypoxic due to capillary occlusion resulting from excess collagen and endothelial cells [7]. Fibroblasts at the margins are more metabolically active, invasive, and capable of activating angiogenesis, presumably for maintaining their invasiveness [8]. Therefore, models utilizing spheroid cell culture systems look very attractive.
Transforming growth factor β1 (TGFβ1) is often used to induce the profibrogenic fibroblast phenotype in vitro [9]. This multifunctional protein regulates cellular growth, differentiation, motility, and production of extracellular matrix during normal tissue healing; importantly, increased expression of TGFβ1 can trigger fibrotic skin disorders [10]. TGFβ1 stimulates collagen growth and secretion and induces fibronectin synthesis in keloid fibroblasts [11]. It would be interesting to evaluate the functional response of model cell candidates to TGFβ1.
The aim of this study was to evaluate the feasibility of using telomerized fibroblasts for the in vitro 3D modeling of hypertrophic scars.
METHODS
Cell lines
The study was carried out using normal (NF) and telomerized (Fb-hTERT) human skin fibroblasts. Primary third-passage NF isolated from the foreskin of 35–38-year-old donors were purchased from a cryobank (Perspective; Russia). In order to account for different phenotypic traits of cells obtained from different donors, three cell lines were used.
Fb-hTERT cells were a gift from Prof. Egorov EE. (Engelhardt Institute of Molecular Biology, RAS). They are a HAF-1608 cell culture with the telomerase catalytic subunit gene. Fb-hTERT cells maintain a stable phenotype over up to 200 passages [12].
Culture
The cells were cultured in a complete DMEM/F-12 (Gibco; USA) medium supplemented with 10% FBS (Dia-M; Russia), 1% antibiotic-antimycotic solution by Gibco, USA (final concentrations: 100 un/ml penicillin, 0.25 μg/ml amphotericin B, 100 un/ml streptomycin) and 1% GlutaMAX solution by Gibco, USA (the final concentration of L-alanyl-L-glutamine was 2 mM).
MMT-based analysis of TGFβ1 effect on cells
For the МТТ assay [13], 2,500 NF cells at passage 4 and Fb-hTERT cells harvested at 70% confluency from the culture flask were plated in each well of a flat-bottom 96-well plate and incubated for 24 h in a complete DMEM/F-12 medium at 37 °С and 5% СО2. Then, the medium was replaced with a fresh medium containing TGFβ1 (ProSpec; Israel), and the cells were incubated for another 48 h, the medium being changed every 24 h. After that, the medium was collected and the wells were washed in DPBS (Gibco; USA); 100 μl of 1 mg/ml МТТ solution (Dia-M; Russia) was added to each well, and the cells were incubated for 2.5 h at 37 °С and 5% СО2. After 2.5 h of incubation, the MTT solution was replaced with DMSO (Biolot; Russia) and the mixture was further incubated at room temperature until formazan crystals were completely solubilized. Absorbance was measured in each well at 490 nm; background absorbance at 655 nm was subtracted. The МТТ assay was run in 3 replicates (5 wells per replicate) using the following TGFβ1 concentrations: 0 ng/ml; 0.1 ng/ml; 1 ng/ml; 5 ng/ml; 10 ng/ml.
Cell metabolic activity was calculated using the formula: 100% * (AB490 (experimental wells) — AB490 (medium))/(AB655 (control wells) — AB655 (medium)). AB stands for absorbance.
Spheroid cell culture
Spheroids were grown in ultra-low attachment 96-well plates (Corning; USA); 5, 10 or 20 thousand NF or Fb-hTERT cells at 70% confluency were dispensed into the wells and incubated for 9 days in a complete DMEM/F-12 medium at 37 °С and 5% СО2. The medium was replaced every 24 h.
To study the effect exerted by TGFβ1 on spheroids, 20,000 NF cells and 10,000 Fb-hTERT cells (sufficient for growing spheroids of comparable sizes) harvested at 70% confluency from culture flasks were dispensed into each well and incubated for 3 days in a complete DMEM/F-12 medium supplemented with 1 ng/ml TGFβ1 at 37 °С and 5% СО2. The medium was replaced every 24 h to maintain stable concentrations of the growth factor.
Spheroids were photographed every day using a Primovert microscope (Carl Zeiss; Germany). Their diameters were measured using ImageJ software and a Fiji extension (National Institutes of Health; USA) [14].
Estimating wound closure rate in cell monolayer using scratch assay
Harvested at 70% confluency from a culture flask, 50,000 NF or Fb-hTERT cells were added to each well of a 24-well plate and incubated for 24 h in a complete DMEM/F-12 medium at 37 °С and 5% СО2 until the monolayer was formed. Then the medium was replaced, and a scratch was made on the cell monolayer with a 1 ml-pipet tip. The cells were washed in DPBS; then, DMEM/F-12 (control) or DMEM/F-12 containing 1 ng/ml TGFβ1 (experiment) was added to the cells. The cells were incubated for 2 days. In each well, the entire length of the scratch was photographed daily. The photos were processed in CellProfiler (Broad Institute of Harvard and MIT; USA) [15]. The closed area of the gap was calculated at 24 and 48 h; the ratio of the closing gap area to the initial scratch area was calculated. The experiment was conducted in 3 replicates in 3 wells per replicate.
Measuring expression of genes associated with abnormal fibrous growth using qRT-PCR
After analyzing the literature, we chose the PAI-1 gene and the genes encoding synthesis of collagen I, collagen III and fibronectin as target genes. Obviously, this list is not exhaustive but the increased expression of these genes suggests modulation of profibrogenic cell potential.
Briefly, qRT-PCR was performed as described below. The suspension of 200,000 cells in 5 ml of the culture medium was transferred to a flask and preincubated for 48 h in a complete DMEM/F-12 medium at 37 °С and 5% СО2. Then, the medium was replaced with a fresh medium containing 1 ng/ml TGFβ1 and the cells were incubated for another 48. The medium was replaced every 24 h. After that, RNA was isolated from the cells using an RNeasy Mini Kit (Qiagen; Germany) according to the standard protocol. The obtained RNA was quantified on a NanoDrop 2000c spectrophotometer (Thermo Scientific; USA). Reverse transcription was performed following the standard protocol using an MMLV RT kit (Evrogen; Russia) and 1 μg of RNA. qPCR was performed using a qPCRmix-HS SYBR+LowROX reaction mix (Evrogen; Russia). For each gene and each sample, the reaction was run in 3 replicates. GAPDH was chosen as a reference gene.
Primers are listed below: GAPDH Forward Primer F: 5'-TCGACAGTCAGCCGCATCTTCTTT-3' Reverse Primer R: 5'-ACCAAATCCGTTGACTCCGACCTT-3'; COL1A1 F: 5'-CCAAGAGGAAGGCCAAGTC-3' R: 5'-ACACGTCTCGGTCATGGTA-3'; COL3A1 F: 5'-CTGGTGCTAAGGGTGAAGTT-3' R: 5'-GTCCAGGTTCTCCTCTTTGTC-3'; FN1 F: 5'-GAATAAGCTGTACCATCGCAAAC-3' R: 5'-ACCAAGACACACACACTCTAAC-3'; PAI-1 F: 5'-GGCTGACTTCACGAGTCTTT-3' R: 5'-CGTTCACCTCGATCTTCACTT-3';
Measuring collagen I production by spheroid culture
Spheroids were grown as described above. After 3 days of incubation, spheroids grown from 10,000 cells were fixed in 4% formalin and embedded in paraffin following the standard protocol. Paraffin sections were stained with goat anti-human collagen I primary antibodies (IMTEK; Russia) and donkey anti-goat IgG FITC secondary antibodies (ab6881, Abcam; USA). Three spheroid samples per each cell line were chosen for the analysis; for further staining, we used 5 paraffin sections per sample. All sections were stained simultaneously using the same reagent kit (antibody solutions and buffers).
The stained paraffin sections were examined under a laser confocal LSM 710 microscope (Carl Zeiss; Germany); in all cases, the settings were identical. Photographs were processed in CellProfiler [15]. For each sample, the mean fluorescence intensity was calculated as the total fluorescence intensity divided by the spheroid area. Mean fluorescence intensity values were normalized to the mean fluorescence intensity of NF cells that had not been exposed to TGFβ1.
Statistical analysis
The obtained data were processed using the programming language R. Differences between the groups were tested using Student’s t test with the Benjamini–Hochberg procedure for multiple comparisons. Differences were considered significant at p < 0.05. Statistical data are presented below as M ± m unless specified otherwise.
RESULTS
Normal and immortalized skin fibroblasts differed in their size and proliferative activity. The surface area of NF cells cultured at low density (> 50% confluency) was 723 ± 54 μm2 (fig. 1А).
Doubling time was calculated by the formula DT = T * ln2 / ln (X1/X0), where Т is incubation time, Х1 is the number of cells after culture, Х0 is the initial number of cells. For NF cells, doubling time was 3.1 ± 0.6 days. Cultured as a monolayer, NF cells reached 100% confluence at a density of 16.5 ± 3.1 * 103 cells/cm2.
The surface area of Fb-hTERT cells was smaller: 675 ± 29 μm2 (fig. 1А). Doubling time was 1.8 ± 0.4 days. Fb-hTERT cells reached 100% confluence at 41.9 ± 7.2 * 103 cells/cm2.
The МТТ assay revealed a dose-dependent effect of TGFβ1 on NF and Fb-hTERT cultures (fig. 1B). The metabolic activity of Fb-hTERT and NF increased significantly after the cells were treated with 0.1 ng/ml and 1 ng/ml of TGFβ1, respectively. For both cell lines, a peak in the metabolic activity was observed following exposure to 1 ng/ml of TGFβ1 and was significantly higher for Fb-hTERT cells (179 ± 12% vs. 135 ± 13%, respectively; p < 0.05). A further increase in TGFβ1 concentrations added to the culture medium caused a decline in the metabolic activity of the cells relative to the effect exerted by 1 ng/ml TGFβ1. Therefore, we decided to use 1 ng/ml TGFβ1 to boost cell profibrogenic activity in the subsequent experiments.
The scratch assay demonstrated that the gap closure rate was different for the NF and Fb-hTERT cells and in the presence of TGFβ1 (fig. 2).
The gap closure rate at 24 h and 48 h after creating the scratch was significantly higher in the monolayer of the control NF cells (in the absence of TGFβ1) than in the control Fb-hTERT cells. At the same time, the gap closure rate did not differ significantly between the NF cells cultured in the presence of TGFβ1 and the control cells. Stimulated by TGFβ1, the Fb-hTERT monolayer closed the scratch two times faster than the control cells at both time checkpoints: 24 h (33 ± 8% and 13 ± 7% respectively) and 48 h (61 ± 10% and 30 ± 7%, respectively). Thus, in the absence of TGFβ1, NF cells closed the scratch in the monolayer faster than Fb-hTERT cells, whereas in the presence of TGFβ1, the Fb-hTERT monolayer recovered faster than the NF monolayer.
Spheroids were grown from 5,10 and 20 thousand fibroblast cells. In all cases, spheroids were shrinking in size during the first 5 days of culture. After that, their size remained relatively stable (fig. 3А). The more cells were used to grow a spheroid, the more pronounced was the rate of shrinking, for both NF and Fb-hTERT cells.
Fb-hTERT spheroids were approximately two times larger than NF spheroids although Fb-hTERT cells themselves were smaller than NF.
TGFβ1 effects on NF and Fb-hTERT spheroids were studied using cellular aggregates formed by 20,000 (NF) and 10,000 cells (Fb-hTERT) (fig. 3B). The sizes of the grown spheroids were comparable. The size is a crucial parameter for cell aggregates since it determines nutrient availability at the margin and in the center and affects the intensity of hypoxia in the center of the spheroid.
Spheroids grown from NF significantly increased in size 24 h after the culture medium was supplemented with 1 ng/ml TGFβ1, as compared with the control NF spheroids (fig. 4).
After that, the differences started to level out and disappeared by day 3 of culture.
Spheroids grown from Fb-hTERT cells displayed a different behavior. In the presence of TGFβ1, they significantly diminished in size on days 1 and 2 of culture, in comparison with the control. By day 3, these differences smoothed out.
Slight differences in the size dynamics of spheroids formed from NF and Fb-hTERT cells at the early stages of the culture exposed to TGFβ1 might be explained by the phenotypic traits of the used cell lines and differences in their initial number. However, by day 3, as the spheroids grew and the cell density increased, there were almost no differences.
NF and Fb-hTERT cells differed in the expression of genes associated with fibrous tissue growth both in the absence or presence of TGFβ1 (fig. 5).
In the absence of TGFβ1, NF cells tended to increasingly express the genes coding for collagen I and III, unlike Fb-hTERT cells, which expressed PAI-1 slightly more actively. The expression of the gene coding for fibronectin synthesis was comparable between NF and Fb-hTERT.
NF cells incubated for 2 days in the presence of 1 ng/ml TGFβ1 exhibited a tendency for increased expression of all studied genes, in comparison with the control. Significant differences were observed for the gene coding for fibronectin synthesis.
At the same time, no significant changes were observed in the expression of COL1A1, COL3A1, FN1, and PAI-1 in Fb-hTERT cells incubated with TGFβ1.
Those differences between the basal and TGFβ1-stimulated expression of collagen I by NF and Fb-hTERT cells were also present in spheroid cultures (fig. 6А). According to microscopy findings, the level of collagen expression in the spheroids derived from NF was significantly higher than in the spheroids formed from Fb-hTERT cells (fig. 6B). When NF-spheroids were incubated with TGFβ1, there was a 1.7-fold increase in collagen I production, whereas for Fb-hTERT-derived spheroids cultured under the same conditions the increase in the production of collagen I was moderate (1.4-fold).
DISCUSSION
We have been exploring the possibility of stimulating the profibrogenic potential of normal and telomerized human skin fibroblasts (NF and Fb-hTERT, respectively) in vitro. It is known that telomerase is active in fibroblasts isolated from keloid scars [6]. In terms of telomerase activity, keloid fibroblasts resemble immortalized cells; therefore, Fb-hTERT make a promising in vitro candidate model for pathological keloid and hypertrophic scars. At the same time, according to the literature, the phenotype of normal fibroblasts can be modified to approximate the phenotype of cells isolated from pathological scars by using growth factors that play a key role in the pathogenesis of pathologic fibrous growth; one of such growth factors is TGFβ1 [16]. TGFβ1 activates a variety of signal cascades triggering increased cell proliferation, adhesion and migration, as well as extracellular matrix production [17].
Importantly, in vitro models of any tissue type, including normal and fibrous connective tissue of scars, must reproduce its 3D structure ensuring natural cell-cell interactions and mutual influences. Therefore, the phenotypic traits of NF and Fb-hTERT cells were investigated in spheroid cultures. This model is actively used in carcinogenesis research and in the studies evaluating the efficacy of anticancer therapies as tumor microenvironments, nutrient supply, gas exchange and physiology is best reproduced in cellular spheroids [18]. However, prior to our experiment, spheroids had not been used for creating a cell model of a pathologic hypertrophic or keloid scar, which indicates the novelty of our findings.
On the whole, intact Fb-hTERT cells, as compared with NF, had a number of phenotypic traits typical to immortalized cells: a smaller size, a higher doubling rate and a higher rate of confluent monolayer formation, which was, perhaps, determined by their high proliferative activity. This feature of Fb-hTERT cells might underlie their higher (as compared to NF cells) metabolic activity both in the absence or presence of TGFβ1 (according to the МТТ assay results).
An MTT assay allows assessing the metabolic and — arguably — proliferative activity of cells [19]. In this study, we have demonstrated a dose-dependent effect exerted by TGFβ1 on the metabolic activity of NF and Fb-hTERT, which was more pronounced for Fb-hTERT cells. This finding is consistent with other reports of TGFβ1 effects on the proliferative and metabolic activity of fibroblasts [20, 21]. In our study, the peak increase of cell metabolic activity was observed following exposure to 1 ng/ ml TGFβ1 and was more pronounced in Fb-hTERT cells.
The size dynamics of growing spheroids was consistent with other reports [22, 23] of spheroids shrinking in size on the first day of culture and then plateauing gradually (spheroid maturation). Unsurprisingly, our spheroids derived from the initially greater number of cells (especially those grown from 20,000 cells) showed a sharper reduction in size, because the optimal diameter of a cellular spheroid is determined by nutrient supply and adequate gas exchange and ranges from 200 to 500 μm [24].
Spheroids formed from Fb-hTERT cells were significantly larger than NF-derived spheroids, although NF cells are bigger than Fb-hTERT cells. This was probably the result of higher proliferative activity normally observed in telomerized cells. At the same time, we cannot rule out that Fb-hTERT spheroids were less capable of retraction due to weak cell-cell contacts.
Exposure to TGFβ1 decreased the size of Fb-hTERT spheroids and also increased the rate of gap closure (in the scratch test), which was lower in intact Fb-hTERT cells than in NF. Perhaps, TGFβ1 not only stimulated Fb-hTERT proliferation but also promoted their adhesion capacity and facilitated cell cooperation. However, this question requires further investigation.
Importantly, Fb-hTERT cells had other phenotypic traits distinguishing them from normally differentiated fibroblasts. For example, production of basic proteins specific to fibroblasts that are building blocks for connective tissue fibers (collagen I and III, fibronectin) was lower in Fb-hTERT cells than in NF (both intact or exposed to TGFβ1); this was also true for spheroid cultures, as demonstrated by PCR-based assays and fluorescent staining. Because Fb-hTERT are an immortalized cell line, their proliferation programs seem to be far more active than differentiation programs. Consequently, the expression of collagen genes (characteristic of differentiated connective tissue cells) was low in both intact and TGFβ1-stimulated cells. At the same time, when exposed to TGFβ1, NF cells demonstrated the phenotype of differentiated cells with increased expression of extracellular matrix-related genes.
It is known that the expression of genes involved in the synthesis of connective tissue components is increased in keloid scars. There are reports of increased transcription and translation of collagens I and III and fibronectin in fibrous growths [2]. The abundance of collagen in keloid tissue is explained by the increased expression of PAI-1 not typically seen in normal fibroblasts [25]. Of note, PAI-1 expression in intact Fb-hTERT cells observed in our experiment was higher than in NF.
Besides, the scratch in the monolayer of intact NF cells closed faster than in Fb-hTERT cells. The scratch test is used for the indirect assessment of cell regeneration potential (the ability to close a wound). The gap closure rate depends on the levels of cell proliferation and/or migration. It is known that TGFβ1 promotes wound healing [17]. In our experiments, exposure to TGFβ1 allowed the Fb-hTERT monolayer (but not the NF monolayer) to recover more rapidly. This finding needs to be further analyzed in Fb-hTERT and NF cultures by evaluating the effects of TGFβ1 on cell adhesion and cooperation programs involved in the implementation of cell capacity to migrate.
CONCLUSION
Telomerized fibroblasts have a few phenotypic traits observed in keloid fibroblasts: high proliferative and metabolic activity; the ability to close a gap in the monolayer (the scratch assay) in the presence of TGFβ1; increased, as compared to NF, levels of PAI-1 expression (trending, statistically insignificant). Other important features include their ability to divide infinitely, maintain phenotypic homogeneity at high passage numbers and form cell spheroids. This makes temolerized fibroblasts a promising candidate for the 3D modeling of pathologic scars. However, the expression of proteins associated with extracellular matrix production (COL1A1, COL3A1, FN1) is low in telomerized fibroblasts, including in the presence of TGFβ1, whereas keloid fibroblasts are characterized by overproduction of these proteins. This fact should be accounted for when exploiting Fb-hTERT cells for modeling hypertrophic scars. Further research is needed to study the possibility of activating the expression of extracellular matrix proteins in these cells.