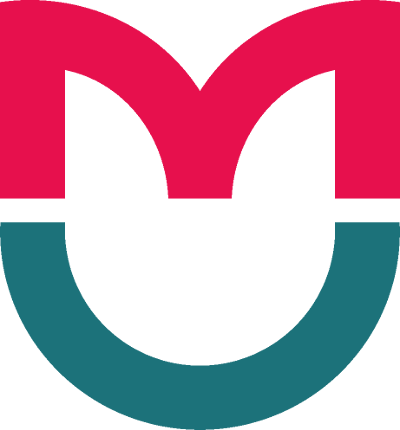
This article is an open access article distributed under the terms and conditions of the Creative Commons Attribution license (CC BY).
ORIGINAL RESEARCH
Detecting reactive oxygen species in biological fluids by platinum nanoelectrode applying amperometric method
1 Lomonosov Moscow State University, Moscow
2 National University of Science and Technology "MISiS", Moscow
3 Medical Nanotechnology LLC, Moscow
4 Helmholtz Institute of Ophthalmology, Moscow
5 Mendeleyev University of Chemical Technology of Russia, Moscow
6 Department of Medicine, Imperial College London, London, United Kingdom
7 WPI Nano Life Science Institute (WPI-NanoLSI), Kanazawa University, Kanazawa, Japan
Correspondence should be addressed: Alexander N. Vaneev
Leninskie gory, 1 bl. 11B, Moscow, 119991; moc.liamg@rdnaskela.veenav
Funding: the study was supported by the Ministry of Education and Science of the Russian Federation in the context of the Agreement # 14.575.21.0147 (project ID RFMEFI57517X0147).
Oxidative stress in tissues is accompanied by excessive generation of reactive oxygen species (ROS) and depletion of the endogenous antioxidants reserves [1]. Entering endogenous metabolic reactions, aerobic cells produce ROS, such as superoxide anion (•O2–), hydrogen peroxide (H2O2), hydroxyl radical (•OH) [2]. Organs of vision are some of the most vulnerable parts of the body [3]. Tissues of the eye are exposed to light for a considerably long time to have radical processes constantly photoinitiated and cells damaged, membrane lipids peroxidized, protein modified through oxidization, DNA damaged through the same process [4]. Many eye pathologies are associated with oxidative stress: cataracts [5], uveitis [6], retinopathy [7], corneal inflammation [8]. All ROS oxidize cellular components, which mostly results in irreversible damage to cells. In the body, antioxidant enzymes (superoxide dismutase (SOD), catalase) and low-molecular antioxidants (vitamins A, C, E, etc.) control concentration of ROS [9].
Thus, learning antioxidant profiles of lacrimal and intraocular fluids is an important task. Modern methods of ROS levels detection are flawed: their sensitivity is low, and, in most cases, the measurements are indirect [10]. The volume of lacrimal fluid a laboratory animal can provide is also limited, which makes the majority of analytical methods inapplicable [11, 12].
Thus, detecting and describing the antioxidant component of lacrimal and intraocular fluids requires special, highly effective and sensitive methods that allow determining ROS level with only a minor volume and concentration of fluids, which is important for ophthalmic diseases diagnosing and prevention [13].
It is common to apply chemiluminescent and spectrophotometric methods [14, 15] to determine the antioxidant activity of lacrimal fluid. These methods imply modeling that allows generating ROS and then assessing the level of antioxidants in the sample based on the model reactions inhibition or stopping. The drawbacks of such modeling systems are the medium's pH, which is different from the physiological conditions, and indirect nature of ROS level measurement process peculiar to the majority of methods available, which imposes a number of limitations and reduces reliability of the data obtained.
Activation of free radicals is one of the causes of vessel neoplasms forming in the context of inflammatory processes. Therefore, oxidative stress is an important constituent of inflammation pathogenesis. Some of the major triggers of inflammation are infections, systemic autoimmune diseases, traumatic eye injuries, burns, hormonal imbalance and metabolic disorders [16, 17]. For example, choroid inflammation, or uveitis, often affects other structures of this organ and translates into various vision disorders [18]. Such disorders result from sensitization of the eye tissues with infection or other antigens and neutrophils penetrating to the nidus. Neutrophils destroy microorganisms and produce ROS by NADPH•H oxidase. Another major source of ROS accompanying inflammation (regardless of the disease etiology) is macrophages [19]. Therefore, antioxidants are a feasible addition to anti-inflammatory drugs in the context of an anti-inflammatory therapy. Complications associated with the inflammatory processes in the eyes mainly result from the activation of free radicals oxidation reactions and accumulation of ROS [3].
In this case, nanoparticles as carries show promise, since they can significantly increase the bioavailability of the drug [20]. SOD nanoparticles of block-ionomer complexes, for example, are non-toxic, non-immunogenic and biocompatible. Due to the repeated reaction with the substrate, antioxidant enzymes are more effective than low-molecular antioxidants. The major advantage of SOD nanoparticles is their ability to circulate in the eye's tissues longer than the native enzymes. In vivo experiments have shown that SOD nanoparticles are quite effective in the context of immunogenic uveitis and chemical eye burn therapies [21].
The goal of this research was to detect ROS in lacrimal and intraocular fluids of rabbits by means of amperometry using a platinum nanoelectrode, as well as to study the effect SOD nanoparticles have on the dynamics of ROS concentration in lacrimal and intraocular fluids of intact rabbits.
METHODS
We obtained SOD nanoparticles following the protocol described in paper [22]. The method implies spontaneous layer-by-layer self-assembly of oppositely charged polyions, which brings stoichiometric complexes with 100% efficiency of loading. To that effect, protamine (Sigma; USA), positively charged at pH = 7.4, was added to a SOD solution in a 0.01 M phosphate buffer PBS (pH = 7.4) (Fermentniye tekhnologii, Russia) mostly negatively charged under physiological conditions; next, a negatively charged block copolymer of polyethylene glycol and poly-L-glutamic acid (Alamanda Polymers; USA) was added, followed by cross-linking with glutaraldehyde (Sigma; USA).
The test subjects were samples of lacrimal and intraocular fluids of rabbits provided by the Helmholtz Institute of Ophthalmology. All in all, 25 chinchilla rabbits (weight 2.0– 2.5 kg each) donated the fluids. They were taken care of in compliance with "Experimental Biological Clinics (Vivaria) Sanitary Management Rules" approved by the Ministry of Health of the USSR on 06.07.73, and order #755 of 12.08.77 issued by the same ministry. Routine care followed the laboratory's SOP. Keeping conditions: indoors, rooms with a 12-hour lighting cycle, temperature range — 18–26 °C, relative humidity of 30–70%, 100% ventilation without recirculation, air exchange rate — 7–12 room volumes per hour, one animal per cage equipped with drinking bowls and feeders. The rabbits were fed «Universal Granulated Compound Feed for Rabbits» (Provimi-Volosovo, Leningrad region).
The pre-tests adaptation period for the animals was 14 days. During that time, their health was monitored for any deviations from the norm. The animals underwent thorough examination before being split into groups. Each animal was given an individual number. Experimental groups consisted of rabbits exhibiting no visible deviations from the normal state of health (randomized selection).
In this study, rabbits of the gray chinchilla line were used as a test system. This line is a regular choice in the context of pharmacological research studying the impact of medicines on the eye tissues.
Gray chinchilla has big eyes, which simplifies evaluation of clinical manifestations of the disease and allows collecting sufficient volumes of fluids from the anterior chamber of the eye.
All operations and activities that involved experimental animals complied with provisions of the order #755 of 12.08.77 issued by the Ministry of Health and the Helsinki declaration. All procedures the animals were part of received approval of the Helmholtz Institute of Ophthalmology ethics committee.All rabbits were simultaneously instilled 30 μl of the nanoparticles solution (2 mg/ml) into one eye, while the other eye served as control. Then, lacrimal and intraocular fluids were taken 10, 30, 60, 120 min after the instillation.
We used a number of filter paper circle patches (diameter 5 mm) to obtain lacrimal fluid samples. The patches were put into the posterior fornix of conjunctiva for 5 min, then taken out and dipped into 300 μl PBS solution for 20 min. The eluate was centrifuged for 10 min at 4000 rpm in a centrifuge (Labsystems; Finland) and the resulting supernatant was used for measurement purposes.
To take the intraocular fluid, we punctured cornea near the sulcus (paracentesis). Once all samples were taken, the animal was euthanized by air embolism.We applied the amperometric method to determine the level of ROS. To this effect, we used platinum-coated carbon nanoelectrodes in the fluids and a silver chloride reference electrode.
Patch-clamp amplifier Model 2400 (AM Systems; USA) allowed registering the potential difference between platinum microelectrode and reference electrode. USB-6211 ADC-DAC converter (National instruments; USA) and WinWCP software allowed data transfer and recording. PatchStar micromanipulator (Scientifica; UK) helped position the nanosensor. The desk for all manipulations was the table of an inverted microscope (Nikon; Japan).
ROS concentration, namely that of H2O2, was evaluated at the potential of +800 mV to the silver chloride electrode [23]. These are the conditions allowing 2Н2О2 ↔ 2Н2О + О2 reaction, which is catalyzed by platinum; since superoxide radical rapidly converts into hydrogen peroxide in the solution, the total concentration of H2O2 determines the general background of the oxidative processes [24]. The level of oxygen was evaluated at the potential of –800 mV.
Quartz capillaries (inner and outer diameters 0.9 mm and 1.2 mm, respectively; fig. 1) were used to make platinum electrodes. The capillaries were pulled by a laser puller (Sutter; USA) to make two nanocapillaries that had a hole with the diameter of 100–500 nm. Pyrolytic carbon was deposited on the nanocapillaries through thermal decomposition of butane in an oxygen-free environment.
Nanocapillary was filled with butane through a rubber tube that fit tightly around the wide end of the quartz nanocapillary. Then, sharp end of the nanocapillary was pushed into a similar quartz capillary of the appropriate size. This capillary carried the laminar flow of argon. Next, starting with the sharp end of the nanocapillary we subjected it to thermal treatment with the help of a butane-propane, distance — 1 cm, duration — 15 s.
We applied the electrochemical cavitation method with subsequent deposition of platinum [25–27]. The electrode was etched in a solution containing 0.1 mM NaOH and 10 mM KCl. Both the nanoelectrode and the reference electrode were immersed in the solution; reference electrode received a symmetrical V-shaped alternating current with an amplitude of 1.5–2 V. Increasing amplitude signaled of the growing current running through the nanoelectrode and, accordingly, of the increasing area of carbon on the quartz tube's tip. When the signal's amplitude was sufficient, the etching was stopped.
After etching in the 0.1 M KCl/10 mM NaOH solution, the treated billets were put into the 2 mM hexachloroplatinic acid (H2PtCl6) solution to electrochemically deposit platinum onto the tip of the capillary, delivering a 0.8 V symmetrical sawtooth-shaped potential to the reference electrode. The differences in cyclic volt-ampere-grams of the nanoelectrode in ferrocenemethanol in the region of negative potentials before and after deposition of platinum indicated the success of the operation, i.e. that we had platinum deposited on the electrode (fig. 2А). OriginPro 8 software (OriginLab, 2018) was used to process the obtained volt-ampere characteristics. We registered the average current values at +800 mV (pro rata to the Н2О2 concentration) relative to the current level at zero potential.
The platinum electrode and the reference electrode, dipped into the samples, allowed measuring Н2О2 level in lacrimal and intraocular fluids taken from the test animals. See fig. 2B for the picture of nanoelectrodes.
RESULTS
Prior to measuring the levels in the samples, we calibrated each platinum electrode using several standard Н2О2 solutions and plotted a calibration curve that consequently allowed determining the level of Н2О2 in the samples (fig. 2С).
According to the calibration, the higher the current value, the greater the concentration of Н2О2 in the sample. Left eye of the test animals was instilled with SOD nanoparticles; right eye, the control, received 0.01 M PBS buffer (pH = 7.4). The data describes each rabbit at 5 different time points: before the instillation (control) and after 10, 30, 60 and 120 min after that. Seemingly due to the individual characteristics of each test animal, the results vary to a certain extent at each time point. In all animals we have seen the Н2О2 level decreasing as the time elapsed from instillation increased.
Because of the considerable variability of the results, we have calculated the difference between Н2О2 levels in test and control eyes of each animal (lacrimal fluid) with the aim to visualize the dynamics of the process as it developed in time. The data obtained were averaged out and presented for 5 rabbits as mean values with standard errors (fig. 3). The values indicate that, compared to the control eye (PBS instillation), the Н2О2 level in the test eye (nanoparticles instillation) was growing for 30 min after instillation. Then, the level of ROS in both eyes became approximately equal.
Next, we measured the ion currents in the intraocular fluid samples. Left eye of the test animals was instilled with SOD nanoparticles; right eye, the control, received 0.01 M PBS buffer (pH = 7.4). After instillation, the Н2О2 level in the intraocular fluid of experimental animals was increasing. As time went by, the values registered were growing increasingly varied at each time point. The data obtained were averaged out and presented for 5 rabbits as mean values with standard errors (fig. 4). Ten min after the instillation was over, the values describing test and control eyes showed no significant differences, but after 30 min the level of Н2О2 in the test eye (nanoparticles instillation) began to grow compared to the control.
DISCUSSION
SOD nanoparticles catalyze reaction 2O2– + 2H3O+ ↔ O2 + H2O2 + 2H2O, which translates into the growing levels of Н2О2 in the samples of lacrimal and intraocular fluids. In the lacrimal fluid, the level of Н2О2 increased 10 and 30 min after the beginning of instillation and returned to baseline an hour after (dome-shaped graph). This is different from the ROS level dynamics seen in the intraocular samples, where the concentration of Н2О2 starts growing only 30 min after instillation. It is difficult for SOD to penetrate to the intraocular fluid, which explains the latent period of about 30 min.
When the SOD concentration in the lacrimal fluid grows, that of superoxide radicals decreases significantly, while the concentration of Н2О2 increases (fig. 3). The Н2О2 concentration reaches the maximum level in 30 min, which indicates a shift in the equilibrium of superoxide radical dismutation reaction towards generation of Н2О2. In 1 hour, the Н2О2 concentration returns to baseline because of the two related processes seen in lacrimal fluid: firstly, in 1 hour the concentration of SOD nanoparticles decreases significantly due to leaching from the eye surface, which means the rate of Н2О2 generation also slows down; secondly, the antioxidant system found in lacrimal fluid decomposes Н2О2 to water and oxygen.
It should be noted once again that the lifetime of superoxide radicals is only about 10-6 s because of their reactivity. Being a nucleophilic compound, O2– is capable of oxidizing lipoproteins and phospholipids of the membranes, which results in the destruction of cells [28]. In addition to the dismutation reaction that results in Н2О2 generation, superoxide radical is also part of the Haber-Weiss reaction (O2– +H2O2 ↔ OH– + OH• + 1O2). Therefore, it is impossible to have superoxide radicals in the lacrimal fluid samples right after they are taken, so all the measurements are based on the concentration dynamics of Н2О2 relatively stable under physiological conditions.
The concentration in the lacrimal fluid reaches the sufficient level immediately after instillation, but penetrating the intraocular fluid takes some time, which is why the concentration of Н2О2 increases after a slight delay.
Physiological barriers in the eye greatly hinder topical application of conventional drugs; the barriers effectively reduce the concentration of the drug at the site of its administration [29]. Stability of the enzyme absorbed by the cells in the form of nanoparticles increases significantly; presumably, it is the result of stabilization of the enzyme molecule against metabolic degradation and/or lysosomal destruction, which prolongs the time of circulation in the eye tissues compared to the native enzyme [30].
In the future, it is possible to measure the level of Н2О2 directly in the eye tissues with the help of nanoelectrodes. Since nanoelectrodes are extremely small (100–500 nm), they can be used to penetrate both isolated cells and live eye tissues for real-time measurements.
CONCLUSIONS
Test making use of platinum nanoelectrode may be of diagnostic value in assessing the course of eye pathologies associated with inflammatory processes. Evaluation of the level of Н2О2 by means of the method suggested allows justified application of the antioxidant drugs in the context of eye inflammation therapy. The technology is simple and sufficiently sensitive, and its effectiveness makes it very promising for biomedical applications. In the context of our research we have shown a method to make sensitive platinum nanoelectrodes that can be used to detect ROS in biological fluids. We have come to the conclusion that this direct method is more sensitive and promising in biological applied research.