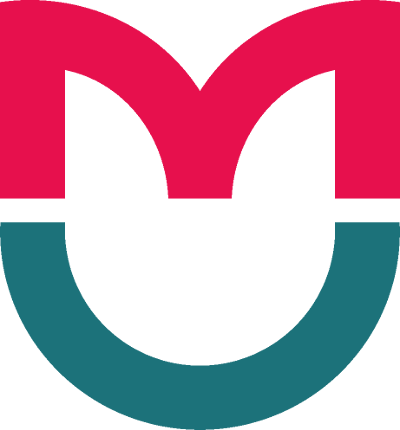
This article is an open access article distributed under the terms and conditions of the Creative Commons Attribution license (CC BY).
ORIGINAL RESEARCH
Molecular origin of surface-enhanced Raman spectra of E. coli suspensions excited at 532 and 785 nm using silver nanoparticle sols as sers substrates
1 Faculty of Chemistry, Lomonosov Moscow State University
2 Emanuel Institute of Biochemical Physics of the Russian Academy of Sciences, Moscow, Russia
Correspondence should be addressed: Evgeniy G. Evtushenko
Leninskie gory, 1 bl. 3, Moscow, 119991; ur.usm.mehc.emyzne@oknehsutve
Surface-enhanced Raman spectroscopy (SERS) is an optical technique that relies on the amplification of the weak Raman signal emitted by a molecule located in close proximity to a metal surface with nanoscale roughness. Dating back a few decades, SERS still has not lost its appeal as a powerful detection technique. It is rapid and simple in instrumentation; it can be optimized to achieve a very high sensitivity and measure multiple analytes. SERS can also be employed for local analysis. Metal nanostructures used for signal enhancement are referred to as SERS substrates and fall into two major categories: nanostructures on solid supports and colloidal sols of metal nanoparticles.
SER spectra of bacteria were first recorded at a 514.5 nm laser excitation wavelength (EW) from Escherichia coli and Bacillus megaterium [1]. It was soon discovered that excitation at 488 or 514.5 nm results in almost identical SER spectra from gram-positive and gram-negative bacteria, as well as their isolated cell membranes: all acquired spectra originated from reduced or oxidized riboflavin (RF) [2–4]. The RF extinction band overlaps with 488 and 514.5 nm EWs, which induces resonant enhancement of the RF spectrum [3]. RF is a component of cofactors of redox enzymes and electron transport proteins found in cell membranes. If a bacterial cell is applied onto the surface of a solid SERS substrate or, alternatively, metal nanoparticles are synthesized or absorbed onto the cell, RF will come to occur in close proximity to the metal surface [3, 4]. From a bioanalytical standpoint, it means that laser sources with short wavelengths of 488 and 514.5 nm cannot be used for the identification of or discrimination between different bacteria.
At the same time, bacterial cells excited at long incident EW (785 nm) have SER spectra that do not contain the bands characteristic of riboflavin. Moreover, SER spectra vary between bacterial species and sometimes strains, not to mention intact and inactivated samples of the same strain [5–7]. This inspired a hypothesis in the early 21st century about the feasibility of SERS for the identification of bacterial species and strains excited at 785 nm wavelength. If adopted, this approach would have sped up pathogen detection in patients’ samples, food products, and environmental objects. However, the molecular origin of bacterial SER spectra at long EWs was vague. According to a proposed hypothesis, such spectra could originate from the molecules localized in the bacterial glycocalyx (a slime layer or a capsule) or bacterial envelope (a cell wall or a membrane), as was the case with short wavelength lasers. Some authors speculated that the spectra might originate from N-acetyl-D-glucosamine [6], amino acid residues, peptides, protein prosthetic groups, phospholipids, metabolites (such as glucose or acetoacetic acid), or DNA and its constituents (guanine and adenine) [5, 7–13]. However, the proposed hypotheses lacked substance: they interpreted the origin of individual bands only ignoring the full spectra. As a result, this area of science was long dominated by a formal mathematical approach that combined the method of principal component analysis (PCA) used to reduce the dimensionality of experimental data and discriminant or cluster analysis aimed to prove the feasibility of discrimination between bacterial genera, species and strains based on their SER spectra [6, 12–15].
But then a study published in 2016 demonstrated convincingly that it was 6 purine metabolites released into the medium by the bacterial cell that were the source of SER spectra at 785 nm EW for 10 investigated samples [16]. The purine derivatives included adenine, guanine, adenosine monophosphate, hypoxanthine, xanthine, and uric acid. The suggested interpretation imposes a dramatic limitation on the use of bacterial SER spectra for the identification/ discrimination of pathogens because the differences between their spectra are caused by only 6 secreted purine derivatives and not the whole diversity of molecules on the cell surface. For example, a hypoxanthine-free E. coli mutant with a silent adenosine deaminase gene was closer in its SER spectrum to Staphylococcus aureus than to the parent strain. The EW used in that experiment was 785 nm. Aggregated gold nanoparticles grown on a solid surface and coated with a thin silica layer were used as a SERS substrate. The aim of our study was to verify the authors’ conclusions using a principally different type of SERS substrates (silver nanoparticle sols) and to investigate the molecular origin of bacterial SER spectra at 532 nm EW lying between riboflavin-dominated (488 and 514.5 nm) and infrared (785 and 1,064 nm) spectral regions.
METHODS
E. coli DH5α (Thermo Fisher Scientific; USA) was used as a model strain. The cells were cultivated in a liquid culture medium consisting of 10.0 g/l tryptone (Difco; USA), 5.0 g/l yeast extract (Difco; USA) and 10.0 g/l chemically pure NaCl (рН 6.8) (Chimmed; Russia) at 37 °C for 14–16 h until the stationary phase was reached. According to the literature [17], the cultured cells should be washed thoroughly to remove the residual components of the culture medium. Bearing that in mind, we applied the following protocol. Briefly, the cells were pelleted in the Beckman J-2-21 centrifuge (Beckman Coulter; USA) at 8,000 rpm for 7 min. The pellet was washed in an equivalent volume of 0.9% NaCl. The procedure was repeated twice. The obtained biomass was diluted with 0.9% NaСl taken at a volume sufficient for obtaining a suspension of 1 • 108 cells per ml. The final concentration was determined spectrophotometrically at 540 nm.
For the experiments with partially inactivated bacteria, the suspensions were placed into a water bath preheated to 70 °С or 90 °С and kept at this temperature for 1 h. The degree of inactivation was inferred from the concentration of intracellular ATP measured by the luciferase-luciferin assay using the reagent kit and calibration standards by Lumtek; Russia.
A sol of Ag nanoparticles (AgNPs) was used as SERS substrate. The sol was prepared by reducing silver nitrate with hydroxylamine hydrochloride in the presence of sodium hydroxide using AgNO3 (ASC reagent, ≥ 99.0%; Sigma-Aldrich; USA), NH2OH•HCl (purified; Prime Chemicals Group; Russia), and NaOH (reagent grade; Mosreaktiv; Russia). Following the original protocol [18], the silver nitrate solution was poured into the alkaline hydroxylamine solution. The final concentrations of the reagents in the mixture were 1 mM AgNO3, 1.5 mM NH2OH•HCl, and 3 mM NaOH. Sols older than 3 days were not used in the experiment.
The absorption spectra of the synthesized nanoparticles were measured in a UV-visible region (300–750 nm) with the cuvette spectrophotometer UV-1800 (Shimadzu; Japan). The size and concentration of AgNPs were measured by nanoparticle tracking analysis (NTA) using the Nanosight LM10 HS-BF system (Nanosight Ltd; UK).
The SER spectra of both intact and inactivated bacteria were recorded on the day of sample preparation. Until then, the samples were stored at +4 °С. Immediately before the measurement, a sample aliquot was centrifuged twice at 3,700 rpm for 5 min in the Biofuge A centrifuge (Heraeus Sepatech; Germany) and washed in an equivalent volume of deionized water. The obtained cell suspension in water was mixed with the AgNP sol at a ratio of 1:1 and incubated for 1 min. Then, the NaCl solution taken at a final concentration of 40 mM was introduced into the mixture to stimulate particle aggregation and enhance the signal. An aliquot of this mixture (260 μl) was transferred into a well of an aluminum well-plate to minimize the background signal and improve heat dissipation. The spectra were measured in 3 to 4 replicates per sample; the samples were stirred by pipetting between measurements.
To study the changes in the SER spectra over time, 5 ml of the E. coli suspension were transferred to deionized water following the procedure described above. The obtained water suspension was stored at +4 °С and its aliquots were picked to register SER spectra over the course of 4 h.The filtrate was prepared by filtering the E. coli water suspension slowly using a syringe filter SFNY030022S (Membrane Solutions; USA) with a diameter of 30 mm and a pore size of 0.22 μm.
At 785 nm EW, SER spectra were recorded using the innoRam BWS445(B)-785S spectrometer (BWTek; USA) with a 785 nm diode laser source and a ×20 PL L 20/0.40 objective. The instrument was operated at a measuring range of 64–3,011 cm-1 and resolution of 4 cm-1. The spectra were recorded using the incident beam power of 42 mW, 5 s signal accumulation time, and averaging over 20 repeated scans. At 532 nm EW, SER spectra were recorded using the iRaman BWS415-532S spectrometer (BWTek; USA) with a 532 nm diode laser source and a ×20 PL L 20/0.40 objective. The instrument was operated at a measuring range of 174–4,001 cm-1 and resolution of 4 cm-1. The spectra were recorded using the incident beam power of 20 mW; 5 s signal accumulation time, and averaging over 20 repeated scans.
The recorded spectra were processed in OPUS 7.0 (Bruker Optik GmbH; Germany). The data outside the 500–1,800 cm-1 range was discarded, and the baseline was subtracted using the Background correction tool. Smoothing was not applied to determine peak positions and intensity. However, SERS data for plots was smoothened using the Smooth tool with a frame width of 9 cm-1. The spectra were processed using vector normalization for a clear visual representation of qualitative differences. Normalization was not performed when the intensities of the spectra were compared.
RESULTS
The popular hydroxylamine technique for the synthesis of AgNP sols [18] is simple and reproducible; the sols it yields significantly enhance the spectra emitted by various analytes, including bacterial cells [19–21]. The AgNP sols we prepared were transparent, deep yellowish-brown in color and did not contain any precipitate. They had a broad and intense absorption band in the near UV-blue region corresponding to the localized surface plasmon resonance of AgNPs with a maximum at 407–409 nm and absorption at this wavelength ranging from 16.5 to 18 (this accounts for 30-fold dilution with deionized water). The number-weighted mean hydrodynamic diameter of the particles measured by nanoparticle tracking analysis was 43 ± 2 nm in three independent AgNP batches. The total particle concentration was (8.0 ± 1.7) • 1011 particles per ml. The synthesized AgNS sols aggregated in 40 mM NaCl did not have their own SER spectra at both EW except for a broad low-intensity band contributed by aluminum (the material of the plate) in the region between 1,200 and 1,700 cm-1. This band can be totally subtracted during data processing.
The reproducibility of intact E. coli SER spectra at 785 nm EW was tested in a series of different experiment. First, we repeatedly measured the spectra of the same mixture of E. coli + AgNPs + NaCl. Second, we measured the spectra of different aliquots of bacterial sample using the same and different AgNP batches. Third, we measured the spectra of independently cultured and isolated E. coli applied onto one and the same AgNP substrate. Repeatedly measured bacterial samples demonstrated good repeatability (fig. 1А). The SER spectra of independently cultured bacterial samples varied considerably (fig. 1B). The most significant variations were observed in the following spectral regions: 508–532; 655; 730–734; 958; 1,450; 1,570–1,576 cm-1.
E. coli stored in water at +4 °С for 4 h (fig. 1C) demonstrated a gradual increase in the total intensity of the SER spectrum over time accompanied by a change in the intensity ratio of its individual bands. For example, the ratio I730 / I655 = 1.2 remained constant at all time points, but the ratio I1325 / I655 monotonously declined from its initial value of 1.6 to 1.0 over the course of 4 h.
The spectra of the intact E. coli suspension in water were compared to its filtrate (0.22 μm) in order to locate the molecules giving rise to the SER spectra (fig. 1D). Considering the slow dynamics, the spectra of aliquots of the initial bacterial suspension were recorded before and after filtration. All spectral bands observed for the cell suspension were present in the spectra of the filtrate. Moreover, the total intensity of the filtrate spectrum was significantly higher.
The SER spectra of intact cells and those inactivated at 70 °С or 90 °С for 60 min were compared in an attempt to understand whether the observed SER spectra can indicate the presence of viable E. coli or whether they come from an inactivated bacterial biomass (fig. 2). The residual concentration of intracellular ATP was also measured in all three sample types (intact bacteria and cells inactivated at 70 °С and 90 °С) as it is indicative of cell viability. The ATP concentrations were 1 • 10-9, 5.6 • 10-12 and 4.1 • 10-12 mol per 1 ml of cell suspension, respectively. On the whole, considerable variability was observed in the number and position of spectral bands. However, the total intensity of the spectrum tended to decrease. The spectra of inactivated bacteria (90 °C) contained only 4 very low-intensity bands characteristic of intact E. coli (730; 1,002; 1,325, and 1,450 cm-1) and two low-intensity bands of the amide III (1,230–1,270 cm-1) and amide I (1,640–1.680 cm-1) regions.
The reproducibility of the SER spectra of intact E. coli was also tested at 532 nm EW. In this case, the intensity of the spectra was twice as high as that observed at 785 nm, resulting in a higher number of informative spectral bands and a better accuracy in locating their position. Similar to 785 nm EW, at 532 nm the high repeatability of the spectra was observed for one and the same aliquot of one and the same bacterial sample (fig. 3A). But the SER spectra of independently cultured and isolated bacteria varied considerably (fig. 3B).
DISCUSSION
Measurements conducted at EW 785 nm demonstrate that the SER spectra of E. coli stored in water are not determined by a single compound, but rather by a mixture of a few different components. This becomes clear when we look at the array of all recorded spectra that contains a fixed set of spectral bands (see the Table). The ratios of the mixture components slowly change over time when cells are stored in water (fig. 1C) and differ significantly between independently cultured batches of intact cells (fig. 1B). Comparison of the spectra of the intact bacterial preparation and its filtrate (fig. 1D) shows that the components of the mixture do not originate from the cell surface but are present in the solution. Moreover, the cell itself can be seen as interfering with the recording of SER spectra, as it adsorbs particles on its surface. This is suggested by a significant increase in the total intensity of the filtrate spectrum in comparison with that of the cell suspension.
Inactivation of bacterial cells demonstrates that the mixture of the compounds in question bears connection to cell viability (fig. 2) but is not a product of passive desorption from the surface of inactivated cells.
The Table features a list of spectral bands observed in all acquired SER spectra of intact E. coli, including the filtrates.
Upon analyzing the literature, we concluded that at 785 nm EW almost all spectral bands are a product of superposition of spectra originating from 4 purine derivatives (adenine, guanine, hypoxanthine, and xanthine). This conclusion is consistent with [16]. Besides, for every individual SER spectrum, the intensity and positions of bands correspond to such superposition as well. (fig. 4А). Unlike the authors of [16] who exploited aggregated gold nanoparticles on a solid surface, we used sols of silver nanoparticles. Considering the possibility of slight variations in the relative intensity of the spectral bands associated with the use of different SER substrates and an increase in intensity following overlay of spectral bands of individual compounds, our description is quite accurate.
Only 3 low-intensity bands remain uncharacterized: 838– 842, 1,002–1,008 and 1,508 cm-1. On the one hand, there is a chance that low-intensity bands can be lost during digital conversion of the spectra from literature sources. On the other hands, it is possible that those bands have never been present in the spectra of individual purine derivatives. Then, their origin can be explained by two hypotheses. First, the bands can result from the interactions between the components of the mixture. For example, a SER spectrum of a mixture consisting of adenine, hypoxanthine and xanthine contains two bands (1,000 and 1,510 cm-1) absent in the individual spectra of its constituents [22]. Besides, the presence of 838–842 and 1,002 cm-1 bands can be explained by minor presence of tyrosine (the most intense spectral bands are 824; 847; 928; 1,046; 1,389 and 1,583 cm-1 [23]) and phenylalanine (the most intense spectral bands are 930; 1,002; 1,031; 1,394; and 1,602 cm-1 [23]).
The suggested origin of bacterial SER spectra explains a considerable variation in the position of peaks observed for some bands within E. coli spectra. Thus, the variability in the position of the peak of a broad multicomponent band ranging from 502 to 574 cm-1 can be explained by an overlap of the following bands: xanthine (508 cm-1), guanine (526 cm-1), hypoxanthine (550 cm-1), adenine (558 cm-1), and guanine (577 cm-1). For the band in the region between 653 and 667 cm-1, the contributing bands are 652 cm-1 (adenine), 657 cm-1 (xanthine) and 667 cm-1 (guanine); for the 724–735 cm-1 band, 725 cm-1 (hypoxanthine) and 734 cm-1 (adenine); for the broad double band with peaks at 1,444–1,452 cm-1 and 1,464– 1,473 cm-1, the contribution is made by guanine (1447 cm-1), adenine (1455 cm-1), hypoxanthine (1456 cm-1), guanine (1466 cm-1), and xanthine (1478 cm-1).
The SER spectra of E. coli are very similar at 785 and 532 nm EW (fig. 4A, 4B) with regards to the position and intensity of some of their constituting bands (fig. 1B, 3B). This finding encouraged us to describe the acquired SER spectra at EW 532 nm as representing a mixture of 4 purine metabolites (adenine, guanine, hypoxanthine, and xanthine) as well. The reference spectra characterized in [16] differ from the acquired E. coli spectra in the type of the SER substrate used and EW, resulting in slight shifts in band positions. Considering that, our description of the experimental SER spectra of E.coli at EW 532 nm can be characterized as satisfactory.
We also explored a possibility of ascribing the bands in the SER spectra of E. coli at 532 nm EW to reduced or oxidized riboflavin and FAD whose reference spectra were borrowed from some early works [4, 24, 25] (fig. 4C). A few high and medium intensity bands of E. coli are absent in the SER spectra of RF including 650, 725–733, 955, and 1,365 cm-1. In turn, the SER spectra of E. coli either miss a number of RF bands or include the bands with a strongly different intensity: 528– 529, 834–839, 1,149–1,156, 1,279–1,289, 1,491–1,502, and 1,523–1,527 cm-1 for oxidized RF and 528, 1,251, 1,501 and 1,530 cm-1 for reduced RF. Nevertheless, the contribution of the RF to the E. coli spectra at 532 nm EW cannot be ruled out. It could additionally increase the intensity of a broad band in the region between 1,300 and 1,350 cm-1 in comparison with the mixture of purine derivatives. However, RF does not dominate the spectrum. This somewhat contradicts the early conclusions about its dominance in the spectra of Pseudomonas aeruginosa, Bacillus subtilis, and Geobacillus stearothermophilus at 532 nm [25]. Such discrepancy can be explained by the difference in the used bacteria species.To sum up, the SER spectra of E. coli at 532 nm EW can be best described as a superposition of the spectra of purine derivatives. Similarly to 785 EW, the variability of the spectra of bacterial samples from different batches at 532 nm EW results from the difference in the concentrations of these compounds released by the cells into the solution.
CONCLUSIONS
The SER spectra of E. coli excited at 785 and 532 nm originate from a mixture of purine derivatives released by the cells into the solution, given that a silver nanoparticle sol synthesized following the hydroxylamine technique is used as a SERS substrate. For both excitation wavelengths, the acquired spectra are best described as originating from adenine, guanine, hypoxanthine, and xanthine. Riboflavin may slightly contribute to the spectra excited at 532 nm. The acquired spectra are characteristic of viable bacteria cells only. Their variability results from the differences in the ratio of the contributing components. Such molecular origin of bacterial SER spectra imposes serious limitations on the use of SERS for bacterial identification and discrimination.