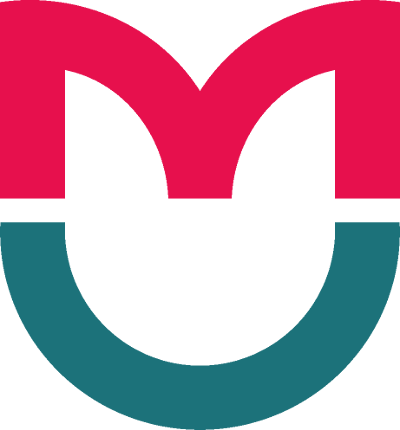
This article is an open access article distributed under the terms and conditions of the Creative Commons Attribution license (CC BY).
ORIGINAL RESEARCH
Lipid metabolic changes in rat brain during permanent cerebral ischemia
1 Shemyakin and Ovchinnikov Institute of Bioorganic Chemistry, Moscow, Russia
2 Faculty of Biology, Lomonosov Moscow State University, Moscow, Russia
3 Lomonosov Moscow State University, Moscow, Russia
4 The Research Institute for Translational Medicine, Pirogov Russian National Research Medical University, Moscow, Russia
Correspondence should be addressed: Dmitry S. Bilan
Miklukho-Maklaya, 16/10, Mosow, 117997; moc.liamg@vosuoleb.dolovesv / moc.liamg@nalib.s.d
Funding: the work was supported by the Russian Science Foundation Grant 17-15-01175.
Author contribution: Bilan DS and Belousov VV were responsible for the study design and drafting the manuscript; Bilan DS, Kotova DA and Kelmanson IV performed surgeries; Kostyuk AI, Kotova DA, Demidovich AD and Panova AS performed biochemical measurements.
According to the World Health Organization (WHO), stroke is the second leading cause of death worldwide. Each year more than 15 million people worldwide suffer stroke. Of these, 6 million die and another 5 million are permanently disabled [1]. The ischemic stroke is caused by angiostenosis or an abrupt blockage of cerebral arteries with resulting reduction incerebral perfusion and rapid damage of brain tissues [2].
Recent studies show biochemical changes occurring in brain cells during ischemia. In particular, hypoxia increases extracellular concentration of glutamate, an important neurotransmitter, and its binding to glutamate receptors [3] leading to a sharp increase of intracellular calcium level. Uncontrolled Ca2+ regulation results in activation of Ca2+ -dependent proteins and upsetting the regulation of cellular cascades [4]. Biochemical changes result in reductions in cell pH and ATP production as well as changes in intracellular ionic ratios [5, 6]. At later stages of pathogenesis, DNA, proteins and lipids are damaged [7]. The pathologic events culminate in mitochondrial dysfunction and extensive cell death [8].
Lipids constitute about half of brain tissue dry weight and have important structural and metabolic functions. Glycerophospholipids and sphingolipids are major components of cellular membranes; cholesterol is a key component of lipid rafts, dynamic assemblies in the cell membrane, regulating membrane motility and signal transduction. Since lipids cannot pass through a blood-brain barrier (BBB), de novo synthesis of lipids occurs in nervous cells. NADPH plays the essential role in the lipid biosynthesis acting a reducing equivalent in anabolic reactions.
Ischemia-reperfusion induces overproduction of reactive oxygen species (ROS) [9] leading to lipid peroxidation. Consequently, there are increased levels of lipid peroxidation products such as malondialdehyde and 4-hydroxynonenal [10] that can be used as markers of cellular oxidative stress.
In response to intracellular ROS increase, antioxidative defense mechanisms are activated in cells. Glutathione (GSH) plays a key role in thiol-disulfide exchange reactions [11, 12] while maintaining the redox state of the glutathione pool is mediated by NADPH-dependent systems [12]. Thus, both parameters are important in the regulation of protection processes against cell damage caused by oxidative stress during ischemia [13].
In our study, we estimated levels of the most important biochemical compounds related to lipid metabolism in rat brain tissues during ischemic damage. Measurements of metabolite concentrations were performed using commercially available kits and standard protocols (MDA, NADP(H)) [14, 15].
METHODS
The study was carried out on male Wistar rats (weight ranging from 280 g to 330 g) obtained from Pushchino breeding facility (Moscow region; Russia). Animals were housed under standard vivarium conditions in plastic cages, three per cage. The animals had free access to food and water. The experimental work (surgeries and biochemical tests) was carried out within a month (June, 2018).
A middle cerebral artery occlusion (MCAO) was performed as described in [16]. Inhalation anesthesia with a mixture of isoflurane with air was used (Aerrane by Baxter; USA): 5% concentration for induction and 1.5% concentration for maintenance of general anesthesia.
The animals were analgesized with 5 mg/kg ketoprofen (Ketonal by Sandoz; Switzerland) administered subcutaneously; local analgesia was induced by administering 2% Novocaine. 0.185 mm MCAO (middle cerebral artery occlusion) sutures were used (403756PK10Re, Doccol; USA).
Tissue sample preparation
The animals were randomized into five groups, four individuals each. The control group included intact animals. In the other four groups, the animals were subjected to permanent right middle cerebral artery occlusion (MCAO). Biochemical analysis was carried out at 5 time points: 1 hour (Group 2), 3 hours (Group 3), 6 hours (Group 4), and 24 hours (Group 5) post-ischemia.
Following 1 to 24 hours of occlusion, the animals were sacrificed and the brains were removed. Brain tissue without cerebellum and olfactory bulbs was frozen immediately in liquid nitrogen and then homogenized thoroughly. The received material was weighed and stored at –70 °C until further processing.
Measurement of FFA level
The quantification of free fatty acids in brain samples was performed with a Free Fatty Acid Quantification Assay Kit (Abcam; Cambridge, UK). At first, the free fatty are being converted to acyl- CoA under acyl-CoA synthetase action. Then specific enzyme oxidizes the obtained monothioesters that is followed by reduction of colourless dye with formation of fluorescent compound (Ex. 535 nanometers; Em. 587 nanometers).
Tissue samples were thawed on ice and then homogenized in 200 μL 1% Triton X-100 in pure chloroform using a manual Potter homogenizer. The obtained homogenate was incubated on ice for 30 minutes to increase the efficiency of extraction. The extracts were centrifuged for 10 minutes at maximum speed, and after that, the organic phase was collected. To remove chloroform, the samples were air dried at 50 °C in a fume hood for an hour and then placed in vacuum dryer for the same time. The obtained lipid fraction was dissolved in 200 μL Fatty Acid Assay Buffer by extensive vortexing.
Aliquots with volumes from 10 to 40 uL were used for the assay. Sample volumes were adjusted to 50 μL with Fatty Acid Assay Buffer solution. Also, a set of palmitic acid standards was prepared for standard curve plotting. 2 μL ACS Reagent (the one with acyl-CoA synthetase activity) was added to each standard and sample well with subsequent incubation at 37 °C for 30 minutes. After that, 50 μL of Reaction mix (45.6 μL Assay Buffer; 0.4 μL Fatty Acid Probe; 2 μL Enzyme Mix; 2 μL Enchancer) were added to each well, with following incubation at 37 °C for 30 minutes.
Fluorescence was measured using Tecan Infinite 200 Pro plate reader (Tecan; Switzerland) at Ex/Em = 535/587 nm. Based on standard readings, the dependence of fluorescence intensity on FFA content in the samples was determined. Based on that, concentrations of FFA in the test samples were calculated. The obtained values were normalized to corresponding tissue weight.
Measurement of cholesterol level
Total cholesterol level (both free cholesterol and cholesteryl esters) in brain samples was quantified using commercially available Cholesterol/Cholesteryl Ester Detection Kit (Abcam; UK) according to the related protocol. In the assay, free cholesterol is oxidized by cholesterol dehydrogenase to generate NADH which reacts with a sensitive probe resulting in strong absorbance at 450 nm.
Tissue samples were thawed on ice and then homogenized in 200 μL CHCl3 : IPA : 10% NP-40 (7 : 11 : 0.1) using a manual Potter homogenizer. Each sample was centrifuged for 10 minutes at maximum speed, and after that, the organic phase was collected. To remove chloroform, the samples were air dried at 50 °C in a fume hood for an hour and then placed in vacuum dryer for the same time. The received lipid fraction was dissolved in 200 μL Cholesterol Assay Buffer by extensive vortexing.
Aliquots with volumes from 10 to 40 uL were used for the assay. Sample volumes were adjusted to 50 μL with Cholesterol Assay Buffer. A set of cholesterol standards was prepared for standard curve plotting. 2 μL Esterase (enzyme that hydrolyzes cholesterol esters to cholesterol) was added to each standard and sample well with following incubation at 37 °C for 30 minutes. After that, 48 μL of Reaction mix (44 μL Cholesterol Assay Buffer, 2 μL Substrate Mix, 2 μL Cholesterol Enzyme Mix) was added to each well, with subsequent incubation at 37 °C for 30 minutes.
The absorbance was measured using Tecan Infinite 200 Pro (Tecan; Switzerland) at 450 nm. Based on standard readings, the dependence of fluorescence intensity on cholesterol content in the samples was determined. After that the concentrations of total cholesterol in the test samples were calculated. The obtained values were normalized to corresponding tissue weight.
Measurement of NADP(H) level
NADP(H) content in brain samples was determined using nitro blue tetrazolium (NBT) and phenazine methosulfate (PMS). Acting as a donor of electrons, NADPH reduces NBT in the presence of PMS converting it into formazan that absorbs at 620 nm. To visualize presence of NADP+, an enzymatic reaction was used where glucose-6-phosphate dehydrogenase (G6PDHs) oxidized glucose-6-phosphate with reduction of cofactor.
About 300 mg of brain tissue were thawed on ice and homogenized using a manual Potter homogenizer in the presence of 1.5 ml of previously cooled carbonate buffer (100 мМ Na2CO3, 20 мМ NaHCO3, 20 мМ nicotinamide). The samples were centrifuged at 4 °C for 5 min at maximum speed. 10 μl were taken out from the supernatant to determine the protein concentration in the solution while the remaining volume was further refined using Amicon® Ultra 0,5 mL 10 K columns (Merck Millipore; Germany) in accordance with the producer’s protocol.
For analysis, the samples were placed to a 96-well plate in an amount of 10 μl/well. A standard series of solutions with known NADP(H) content was prepared. Samples were brought up to 100 μl with a reaction mixture of the following composition (per 10 reactions): 540 μl MQ, 100 μl 1M TRIS-HCl buffer (pH 8.0), 50 μl of 10 mM NBT, 150 μl of 10 mM PMS, 50 μl of a 100 mM EDTA solution, 10 μl of 100 mM glucose-6-phosphate. Samples were incubated at +37 ° C for 5 min, following which the reaction was initiated by adding 1 μl of 250 U/ml glucose- 6-phosphate dehydrogenase.
Absorbance at 620 nm was measured for each sample using Tecan Infinite 200 Pro plate reader (Tecan; Switzerland) in 10 min sessions. Each probe analysis was repeated without enzyme for the determination of background formazan formation under the action of cell lysate components. The dependence of the reaction rate on the content of NADP(H) in a sample was determined. The resulting calibration graph was used to determine the concentration of total NADP(H) in the experimental probes. The values obtained were normalized to the protein concentration.
Measurement of malondialdehyde level
To determine the intensity of lipid peroxidation in the samples, a modified TBARS assay was used. Malondialdehyde, which is one of the key products of lipid peroxidation, reacts with two 2-thiobarbituric acid (TBA) molecules to form a colored compound that intensively absorbs at 532 nm.
Approximately 100 mg of tissue were thawed on ice and homogenized in 750 μl of previously cooled MQ-grade pure water using a manual Potter homogenizer. 5 μl of butylated hydroxytoluene dissolved in methanol at a 10% concentration (w/v) were added to water to prevent ex vivo oxidation. The samples were centrifuged at 3000 × g for 15 min at 4 °C to obtain a soluble fraction containing cell membranes. At this stage, 10 μl were removed from supernatant for further assessing protein concentration. 250 μl of 40% trichloroacetic acid (TCA) were added to 350 μl of samples for precipitation of proteins and stirred vigorously. The samples were again centrifuged at 3000 × g for 15 min at 4 °C.
For analysis, 500 μl supernatant volume was mixed with 500 μl of 0.67% TBA in 0.05 N NaOH. To prepare a standard solution series, 0.5 mM 1,1,3,3,-tetramethoxypropane (TMP) dissolved successively in 1 ml of 96% ethanol and 49 ml of MQ-grade water was used. Standard solutions were mixed with 500 μl of 0.67% TBA in 0.05 N NaOH, after which all samples were incubated at +100 °C for 15 minutes. After the incubation, aliquots of 200 μl were placed in a 96-well plate, and absorbance at 532 nm was measured using Tecan Infinite 200 Pro (Tecan; Switzerland). A calibration graph was created in accordance with the standard series samples, and MDA content was determined accordingly. The values obtained were normalized to the protein concentration.
Measurement of reduced glutathione (GSH) level
GSH in brain samples was quantified using commercially GSH/GSSG Ratio Detection Assay Kit, (Fluorometric-Green Abcam; UK). In the assay, non-fluorescent Thiol Green dye becomes strongly fluorescent upon reacting with GSH (Ex. 490, Em. 520).
Tissue material was thawed on ice and homogenized in 400 μl of phosphate-saline buffer (pH 7.4) containing 0.5% NP-40 using a manual Potter homogenizer. The homogenates were centrifuged at maximum speed for 15 min at +4 °C. At the next stage, the samples were deproteinized by adding 1/5 volume of 100% (w/v) trichloroacetic acid (TCA). After intensive mixing and incubation on ice for 10 min, the samples were again centrifuged at 12,000 × g for 5 min at +4 °C. The TCA was neutralized by adding a NaHCO3 solution to the samples. Finally, the last centrifugation was carried out at 13,000 × g and +4 °C for 15 minutes.
For analysis, the supernatants were diluted by 10 times with Assay Buffer solution, and aliquots of 50 μl of each sample were placed in a 96-well plate. A set of standards with known concentrations of GSH was also prepared. 50 μl of Thiol Green solution were diluted by 100 times with Assay Buffer and then added to samples. The samples were incubated in the dark for one hour.
Fluorescence was measured using Tecan Infinite® 200 PRO (Tecan; Switzerland). Based on standard curve data, dependence of fluorescence intensity on the amount of glutathione in the sample was determined. The calibration graph was used to determine GSH content in each sample. The values obtained were normalized to starting tissue weight.
Measurement of protein concentration
To determine concentration of proteins, a commercially available Bicinchoninic Acid Protein Assay Kit (Sigma-Aldrich; Germany) was used. In each series of experiment, a set of standards with known concentration of bovine serum albumin (BSA) protein was prepared. A mixture containing 1 part of 4% (w/v) copper (II) sulfate pentahydrate and 50 parts of the bicinchononic acid solution was added to each sample. The samples were incubated at +37 °C for half an hour.
Absorbance at 562 nm was measured using Tecan Infinite 200 Pro (Tecan; Switzerland). Using standard curve, protein concentrations were estimated for each sample.
RESULTS
A significant change in NADP(H) pool level was observed in 6 hours after MCAO. For control group, it was 10.64 ± 0.57 µmol/mg of protein while for damaged brain area, it was 4.45 ± 0.79 µmol/mg of protein. In 24 hours after MCAO, NADP(H) level was 1.57 ± 0.46 µmol/mg of protein that is about 7 times lower as compared to control group (figure A).
Since NADP(H) is involved in many biosynthetic processes including the lipid metabolism, we expected to observe a significant decrease in cholesterol and fatty acids levels in brain tissues. In our experiment, the level of total cholesterol (both free cholesterol and cholesteryl esters) began to decrease in 6 h after MCAO: 6.85 ± 0.1 μg/mg of tissue while control value was 7.29 ± 0.20 μg/mg of tissue accordingly. In 24 hours after MCAO, the total cholesterol level in damaged tissues continued to decrease to value of 5.95 ± 0.4 μg/mg of tissue (figure B). For Group 3 (3 hours after MCAO), no difference in cholesterol level was observed as compared to control group. However, since a significant variation of values was observed for animals in Group 3, it is likely that 3 hours after MCAO, the cholesterol level started to decrease in damaged tissues.
The level of the free fatty acids (FFA) in damaged brain tissues differed from related control level in Group 5 (24 hours from MCAO) only: 0.25 ± 0.003 nmol/mg vs 0.31 ± 0.01 nmol/mg (figure C).
Malondialdehyde (MDA) is one of the lipid peroxidation markers. The tissue injury causes a slowdown in cell biosynthetic processes resulting in accumulation of biodegradation products accordingly. In control group, the MDA level was 846.23 ± 63.41 pmol/mg protein showing a normal functional level of cell biodegradation processes. A pathology occurs when cells fail to withstand the damage and maintain proper biosynthesis levels. In 24 hours after MCAO, MDA level in damaged brain tissues increased to 1272.61 ± 124.47 pmol/mg protein (figure D) in ischemic tissue. At this time an extensive cell death occurred that can be visualized by staining of brain slices using specific dye such as 2,3,5-triphenyltetrazolium chloride (TTC).
The glutathione pool is an important redox component in cells. In particular, GSH is used by antioxidant enzymes such as glutathione peroxidase to neutralize ROS. Maintaining optimum GSH/GSSG ratio is essential to cell viability. NADPH is required for the regeneration of the reduced form of glutathione (GSH). In our study, no change was observed in the GSH levels for Groups 2–4 (within 6 hours from MCAO). In 24 hours after MCAO, GSH level in damaged brain tissues decreased to 11.8 μg/mg compared to 35.1 μg/ mg in the control group (figure Е).
Thus, levels of FFA, total cholesterol, GSH and MDA changed significantly in 24 hours after MCAO while the level of NADP(H) was found to decrease in 6 hours after MCAO. X-axis: Time, hours (after MCAO). Error levels correspond to a standard error of an average. 4 animals per group.
DISCUSSION
Cerebral ischemic injury is a stress accompanied by structural damage at molecular or cellular level. Protection of tissues from the effects of ischemic damage occurs in different ways at many levels — through prevention of damage, repair and replacement of damaged structures.
Ischemic stroke is known to be accompanied by excessive and unregulated production of ROS [9] that are highly reactive compounds interacting with various cell compounds. One key target of ROS is lipids — both free and located within cell membranes. Lipid peroxidation is one of the common ways for the interaction of lipids and ROS. The process is a radical chain reaction, the end products of which are covalent aggregates of lipids as well as lipid hydroperoxides and lipid endoperoxides formed in the presence of oxygen [10]. The latter join intramolecular rearrangements with subsequent fragmentation of hydrocarbon tails. It is therefore feasible that an oxidative stress, while in the lipid phase, leads to an increase in the concentration of low molecular weight carbonyl compounds such as malondialdehyde (MDA). Most of the methods aimed at identifying oxidative stress are at least in some way based on registration of compounds of the above mentioned class [17, 18].
Interaction of lipids with reactive oxygen species may lead to a release of free fatty acids. Cell death in the foci of ischemia can also be followed by lipid hydrolysis [19]. It is known that free fatty acids can undergo auto-oxidation. Moreover, the cyclooxygenase pathway in the metabolism of arachidonic acid involves lipid hydroperoxides leading directly to the formation of oxygen radicals [10, 20]. Summing up the above, each and all of the mentioned factors will lead to an even greater contribution to peroxidation.
In practice, in many studies involving patients or laboratory animals, detection of MDA level in blood is performed. It was shown that MDA level increased significantly during the first 24 hours of ischemia, and it remained elevated for up to one week. A correlation of MDA level with a mortality rate has been shown for stroke patients [21]. Assessment of the concentration of MDA in ischemic brain can provide valuable information on the extent of tissue damage [22].
In our study, a model of permanent occlusion of the middle cerebral artery in rats was used. Under given conditions it was possible to register a noticeable increase in the concentration of MDA in 24 hours after MCAO. To better investigate the process, further studies are required involving ischemia/ reperfusion model. Reperfusion is believed to be the key source of ROS generation. Cell death during permanent ischemia may be supposed to take place due to reasons other than lipid peroxidation; presence of dead tissue becomes visible within a few hours after MCAO. In fact, the approach based on TBARS analysis may be subject to artifacts likely involved at the sample preparation stage. Addition of antioxidants like butilated hydroxytoluene reduces the formation of MDA in the presence of atmospheric oxygen, still does not completely eliminates it. Thus, detecting minor shifts of MDA concentration with reference to control probes may be very difficult.
The concentration of reduced glutathione (GSH) was used as one of the markers of the cell redox state. Similar to MDA, we did not detect any changes in GSH level during the active phase of ischemic damage. However, its concentration decreased significantly in 24 hours after MCAO. Since GSH is involved in thiol-disulfide exchange reactions, an increase in ROS level results in an increase of glutathione oxidized form due to activation of antioxidant enzymes. This suggests that a noticeable increase in occurrence of oxidized lipids with subsequent cell death may lead to a significant decrease of GSH level. Still, to confirm this, further investigations are necessary — specifically, using the ischemia/reperfusion model. On the one hand, prolonged peroxidation may lead to a depletion of the GSH pool; on the other hand, the shortage of GSH can drive the system to a state in which peroxidation (which until then could have been restrained) starts causing a damaging effect. Finally, decrease of GSH level may be not due to the functioning of antioxidant systems in membranes. For instance, tissue necrotization is characterized by inability to maintain the GSH pool due to poor functioning of related enzyme systems and the lack of reducing equivalents.
In our study, concentration of NADP(H) decreased by 2 times in the first 6 hours after MCAO and by almost 7 times in 24 hours after MCAO. The NADP(H) decrease is believed to have an adverse impact on the antioxidant systems performance as well as on the anabolic processes. As mentioned earlier, this factor is likely to contribute to the decrease of GSH pool as well as the increase of lipid peroxidation level.
Not all the lipids damaged during peroxidation can be repaired. We believe that during ischemic cerebral damage, anabolic pathways involved in lipid biosynthesis should be activated in order to replace damaged components. The NADP(H) abundance is known to be connected with an efficiency of the biosynthesis of fatty acids and cholesterol as these metabolic pathways require reducing equivalents. As it was mentioned, NADP(H) level changed during the experiment.
In a large number of lab and clinical studies, FFA level in blood plasma was detected as this index can be considered a potential biomarker for detection of early stages of ischemic stroke. Hydrolysis of brain phospholipids with release of FFA into blood stream occurs during a stroke that may be a reason of observing increased FFA level in blood in the first few hours [23]. Based on other research data available, there is a correlation between FFA occurrence and the severity of disorder: the higher the level of FFA, the higher severity level observed [24, 25]. Though changes in FFA profile are of substantial metabolic importance, there is still a lack of data related to brain tissues.
In this work, we detected FFA levels in brain samples at different time points. A significant decrease in FFA level was observed in 24 hours after MCAO; during the acute phase of stroke there was no difference between FFA levels in test groups and the control group. Thus, in our experiment no evidence was received in support of activation of the synthesis of fatty acids for replacement of damaged membrane components. A decrease in FFA level after 24 hours from MCAO is most likely due to cell death leading to depletion of NADP(H) content, disruption of the enzyme system and perhaps some other factors. For the studied model of permanent ischemia in rats, it is likely that no accumulation of lipids hydrolyzed after cell death occurs in brain tissues.
Similar data were obtained with regard to cholesterol concentration however, in case of cholesterol the decrease was observed earlier — in 6 hours after MCAO. In comparison to the original level of metabolites, concentration of cholesterol and FFA decreased similarly in a time lapse of 24 hours after the occlusion (1.24 times for FFA and 1.27 times for cholesterol).
CONCLUSIONS
In the permanent cerebral ischemia experiment, the levels of total cholesterol, FFA, MDA, GSH, and NADP(H) in brain tissues of rats have been measured. It was shown that in 24 hours from the onset of ischemia, there was a significant decrease in levels of FFA, total cholesterol and GSH, and an increase in the level of MDA, a marker of lipid peroxidation. NADP(H) level decreased twice in 6 hours from MCAO.