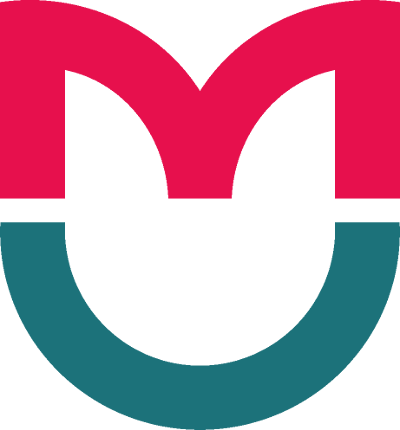
This article is an open access article distributed under the terms and conditions of the Creative Commons Attribution license (CC BY).
ORIGINAL RESEARCH
Synchrotron IR-microspectroscopy-based visualization of molecular and chemical interactions between dental cement, biomimetic composite and native dental tissue
1 Voronezh State University, Voronezh, Russia
2 Burdenko Voronezh State Medical University, Voronezh, Russia
3 Australian Synchrotron, Melbourne, Australia
Correspondence should be addressed: Pavel V. Seredin
Universitetskaya pl.1, Voronezh, 394018; ur.usv.syhp@luap
Funding: the study was supported by the Russian Science Foundation (Grant 16-15-00003).
Acknowledgment: IR microspectroscopy was conducted at the Australian Synchrotron.
Author contribution: Goloshchapov DL planned the study, analyzed the literature, collected and interpreted the obtained data; Kashkarov VM collected, analyzed and interpreted the obtained data; Ippolitov YuA planned the study, prepared the samples, collected and analyzed the data; Ippolitov IYu prepared the samples; Jitraporn Vongsvivut conducted IR microspectroscopy; Seredin PV planned the study, analyzed the literature, collected, analyzed and interpreted the obtained data, and conducted IR microspectroscopy.
Although modern composite materials and bonding resins used in restorative dentistry are durable and have a good adhesive capacity, their chemical and structural resemblance to native hard dental tissue is low [1, 2]. The difference in physical and chemical characteristics between synthetic materials and the natural enamel and dentin of human teeth necessitates development of novel restorative composites [2, 3]. Extensive research effort is being channeled into improving the tooth-material interface by employing innovative bonding systems and hybrid buffer layers that increase the durability of dental restorations and enhance the bonding strength between the filling material and hard dental tissue [3–5]. One of the most important questions that should be addressed by researchers is reliable chemical bonding between dental composite fillers/ bonding agents and the natural tissue of human teeth [6].
So far, biomimetic materials, whose composition and (nano)structure mimic those of hard dental tissue, have offered the best solution to the problem of interface quality between natural enamel/dentin and synthetic adhesives [7–9]. It is a well-known fact that nanocrystalline hydroxyapatite (HA), which is identical in composition and morphology to enamel apatite found in human teeth, facilitates better integration of restorative materials with the tooth [5, 10]. Besides, enrichment of biomimetic agents with polar amino acids occurring in the natural enamel matrix [11–14] creates a possibility for high-quality repair of damaged enamel or dentin layers and improves mechanical properties of the restoration [12, 15].
There are a few methods used to assess integration of the bonding resin with natural dental tissue and the quality of resin-enamel or resin-dentin interfaces. Fourier-transform infrared spectroscopy (FTIR) is a non-destructive technique that provides information about the molecular structure and fine structural properties of biological objects based on the analysis of their fingerprint vibrational IR spectra [16]. FTIR can be applied to study mechanisms of molecular transformations in biomimetic materials [17, 18] and dental adhesive agents [19], analyze the molecular composition of human teeth [6], and register new mineral phases [20]. Microscopes and sources of synchrotron radiation for IR spectroscopy are instrumental in acquiring large arrays of spectra from a microscopic area of a studied biological sample [17]. Based on the acquired IR spectra, chemical images of the sample can be generated, offering a wealth of information about molecular bonds and their spatial distribution.
The aim of this work was to study molecular and chemical properties of an interface between a dental cement, a biomimetic buffer layer and hard dental tissue based on multidimensional visualization of synchrotron IR spectroscopy data.
METHODS
Synthesis of biomimetic material and sample preparation
The biomimetic composite material was fabricated similar to the bonding system described in [21]. The composite contained hyaluronic acid (0.01–0.05 wt. %), L-histidine (0.01–0.2 wt. %), L-lysine hydrochloride (0.05–0.4 wt. %), L-arginine hydrochloride (0.2–1.6 wt. %), ethylene glycol monomethyl ether (30–85 wt. %), diglycidyl dimethacrylate (1–15 wt. %), urethane dimethacrylate (1–15 wt. %), ethanol (2–20 wt. %), and water (the rest). The analysis of optical absorption and emission properties found that the synthesized material, which contained the listed amino acids, was similar in its properties to natural tooth enamel and dentin [22]. Synthetic carbonate-substituted hydroxyapatite (CSHA) was added to the biomimetic buffer (0.01 g of CSHA per 1 ml of the mixture) to improve the similarity of the biocomposite to natural dental tissue [20].
To ensure adhesion of the biomimetic composite to the tooth, we used a universal adhesive agent that effectively bound to the synthesized materials [23]. The adhesive agent was enriched with CSHA (1 ml of the adhesive per 0.01 g CSCA). Hydroxyapatite was combined with the biomimetic buffer and the adhesive agent, and the mixture was subsequently homogenized for 30 seconds using a QSonica Q55 sonicator (QSonica; USA).
Integration of the synthesized biocomposite material with hard dental tissue was tested on the dental specimens obtained from the patients aged 18 to 45 years who had indications for tooth extraction. The specimens were prepared for filling as described below.
First, enamel was removed using an air-driven micromotor hand piece with a round-head tungsten vanadium steel bur operated at 4,000 rpm and a water-cooling system necessary to avoid overheating of the dental matrix. Dentin was spared. Cavity preparation was finished using a low-speed hand piece. The cavity was washed and dried with compressed air.
Second, enamel was etched for 60 s with a 37% phosphoric acid etching gel, rinsed with water and air-dried. Then, a dentin conditioner [21] was applied to the dentin surface for 20–30 s. The conditioner contained hyaluronic acid (0.01–0.05 wt. %), L-histidine (0.01–0.2 wt. %), L-lysine hydrochloride (0.05–0.4 wt. %), and L-arginine hydrochloride (0.2–1.6 wt. %). After that, the cavity was dried.
Third, the biomimetic buffer was evenly applied to the walls of the prepared cavity and dried with compressed air 20 seconds later. The biomimetic layer was coated with a universal CSHA-containing light-curing adhesive agent that was photopolymerized for 20 seconds.
Finally, 1 min after the previous step, the biomimetic buffer layer was coated with a commercial restorative dental compomer containing adhesive components.
Considering the requirements for the geometry of samples subject to IR microspectroscopy, we prepared 7 plane-parallel sections of the restored teeth immediately before the study as described in our previous work [24].
Method of sample analysis
The molecular composition of the samples and the interface between the restorative dental compomer, the biomimetic buffer layer and the native hard tissue of the human tooth was analyzed using attenuated total reflection (ATR) Fourier transform synchrotron infrared microspectroscopy. Measurements were conducted at the Australian Synchrotron Infrared Microspectroscopy Beamline (Melbourne, Australia) equipped with a Hyperion 3000 FTIR microscope (Bruker; USA) and an ATR-FTIR accessory with a germanium prism (Melbourne, Australia) [17]. Figures 1A and B are show the studied segment (marked with the rectangle) that yielded IR absorption spectra at 3800–700 cm–1 wavelength (fig. 1C).
Using the IR microscope and OPUS 7.5 software (Bruker; USA), we acquired a cluster of IR spectra from a small sample area of 100×100 μm in size (fig. 1B) with a 2 μm sampling interval and constructed one-dimensional IR images (maps) based on the color codes for the intensity of IR absorption bands (fig. 1D). The lowest absorbance intensity is shown in red, whereas the highest, in blue. The map demonstrates the distribution of absorbance intensity of a molecular group and, therefore, reveals its concentration in a specified sample area.
RESULTS
By applying IR spectroscopy to the interface between the light-curing adhesive agent, the biomimetic composite material and natural dental enamel/dentin (fig. 1B), we were able to identify the major vibrational modes active in the IR spectrum (fig. 1C) that can be used as spectral fingerprints for the compounds present in the studied sample area.
The absorption band at 1163–981 cm–1 had the highest intensity and represented the PO4 mineral component of enamel/dentin apatite [22]. The range between 1700 and 1100 cm–1 wavelength represented the protein constituents of organic enamel/dentin and the components of the biomimetic buffer (see Synthesis of biomimetic material and sample preparation). Here, the most active vibrational modes were observed for the CH2–CH3 collagen group at 1457 cm–1 and for Amide absorption bands (Amide I at 1650 cm–1, C=O stretching; Amide II at 1550 cm–1, N–H bending, and Amide III at 1245 cm–1, CN stretching) [18, 19, 22]. We also recorded vibrations at 1725 cm–1 wavelength produced by the methyl ester group (–COOCH3), the component of the restorative Bis-GMA dental compomer [19].
fig. 2А features the IR map of РО4 distribution across the studied interface segment (fig. 1B). The IR spectrum specific for this group (Fig 2B) was acquired from the area of enamel-biomimetic layer integration. The spectrum contained vibrations of the РО4 group at 1163–981 cm–1 wavelength occurring in natural enamel /dentin apatite [19, 20] and the biomimetic agent. We concluded that the part of the interface occupied by the restorative compomer did not contain phosphate groups. The zone bordering on tooth enamel where spectral vibrations were observed at 1163–981 cm–1 was ~30 μm in size (fig. 3А, the dotted line).
For further information about the studied interface, an IR image was generated (fig. 3A). This IR map shows the distribution of absorbance intensity for CN, NH, C=O, and CH2/ CH3 in the range between 1718 and 1358 cm–1 wavelength (fig. 3B). The listed molecular groups are components of collagen, Amide I and Amide II (the organic constituents of tooth enamel/dentin added to the biomimetic buffer).
The results of the IR map analysis (fig. 3A) suggest that the distribution of organic components in the biomimetic layer was more homogenous than the distribution of phosphate groups. These findings are consistent with the fact that the proportion of hydroxyapatite in the biomimetic layer was lower than the proportion of organic components.
The IR spectrum presented in fig. 1C contains an absorption band at 1725 cm–1. It is well known that such vibration is typical for dental cements based on Bis-GMA and polymethyl methacrylate and suggests the presence of the ester group (–COOCH3) [19]. Moreover, this spectral band (fig. 4B) does not overlap with other vibrations, which allowed us to visually represent it as an IR image (:media_4А).
The IR map demonstrates spatial distribution of the restorative dental compomer in the analyzed sample segment (fig. 4A). The figure shows that the distribution of absorbance intensity of the ester group (–COOCH3) coincides with the spatial distribution of the dental compomer on the map (fig. 1B).
The analysis of the acquired spectra (fig. 1B) allowed us to identify an intensive absorption band for Amide III at 1269–1224 cm–1 and construct a separate IR map for the biomimetic layer (fig. 5A). Of note, its vibration (fig. 5B) did not overlap with the absorption spectra of other functional groups, meaning that it can be used as a marker of a biomimetic composite.
Comparison of the optical data (fig. 1B) and the IR map (fig. 5A) clearly shows that the molecular group at 1269–1224 cm–1 can be localized to only a narrow segment of the interface between the light-curing material, the biomimetic layer and the enamel/dentin of the human tooth.
DISCUSSION
IR spectroscopy of dental materials [19], biomimetic composites [20], and natural tissue of human teeth [6] characterizes the molecular composition of the studied samples as a single whole. In contrast to the research studies cited in this article, one-dimensional images (IR maps) based on the color codes of absorbance intensity of 4 main spectral ranges (1752–1704 cm–1, 1718–1358 cm–1, 1269–1224 cm–1, and 1163– 981 cm–1) became a good visual representation of the spatial distribution of molecular groups across the studied sample and of molecular-chemical interactions occurring at the interface between the light-curing material, the biomimetic layer and the enamel/dentin of the human tooth.
The IR map of the phosphate group (fig. 2A) revealed the presence of an area with absorbance intensity varying from 1.0 to 7.0. This was part of the biomimetic buffer layer that contained synthetic carbonate-substituted calcium hydroxyapatite introduced into the biomimetic material in order to increase its molecular and chemical resemblance to natural tooth structures [20]. The presence of CSHA in the biomimetic material allowed us to clearly discriminate between the biomimetic layer and the restorative compomer on the IR map (fig. 2A), where color changes were determined by the intensity of vibrational modes representing the РО4 group of CSHA. The margin between the natural dental tissue and the biomimetic composite material was blurred, which proves the high similarity of the composite to natural enamel and dentin.
It should be noted, though, that alone, the IR map of phosphate groups is not enough to study the integration of dental cements with the dental enamel/dentin mediated by the biomimetic buffer layer. At 1163–981 cm–1 the absorption spectra of phosphate groups (:media_2B) can overlap with the vibrational modes of aluminum silicates or silica that are common components of restorative dental materials [19, 25]. However, the analysis of the interface margin (:media_1A and 2A) did not reveal significant amounts of such compounds in the analyzed spectral range (:media_2B), which can suggest their low concentration in the studied sample area.
While the IR map shows the distribution of the phosphate group, the IR image of vibrations at 1718–1358 cm–1 corresponding to the organic components of the sample (:media_3А) helps to accurately identify the margin between enamel and dentin. In :media_3A, the dentin area (shown in red) has a higher organic content than enamel (shown in green), which is consistent with the available reports [20].
At the same time, when studying the interactions between the dental compomer/the biomimetic layer and hard dental tissue, one should bear in mind that the spectral range between 1718 and 1358 cm–1 contains a few overlapping bands [18]. This complicates result interpretation and makes it difficult to draw unambiguous conclusions about the type of interactions at the compomer/buffer/hard tissue interface.
The IR spectra of the ester group (–COOCH3) (fig. 4А) and Amide III (fig. 5A) do not overlap with other vibrational modes. Therefore, the analysis of their IR maps allows us to make the following conclusions. First, it can be clearly seen that the area of integration between the restorative compomer and natural tooth enamel, where absorbance intensity for –COOCH3 varies from its peak to the lowest value, covers a spot ~14 μm in width and overlaps with the area dominated by organic components (fig. 4А, fig. 5А). Second, the analysis of the distribution of absorbance intensity for Amide III (fig. 5А) present in the biomimetic layer suggests that this layer separates hard dental tissue from the dental cement.
All IR images (fig. 2А, fig. 3А, fig. 4А, fig. 5А) offer a good visual representation of the buffer layer, whereas other imaging techniques provide information about the morphology of the tooth-material interface only [2, 4, 15]. This means that buffer layers caught in the integration area and close to each other in composition are hard to analyze.
It is not always possible to visualize interactions occurring at the heterogonous interface between structurally similar materials using a series of one-dimensional IR images [6]. This is a limitation of a one-dimensional approach to the identification of spectral changes for the materials only slightly different in their chemical structure. However, this limitation can be overcome by using multidimensional clusterization methods that can effectively systematize vast arrays of multicomponent IR spectra [26]. Using this approach, we were able to analyze the features of a complex dental compomer/biomimetic layer/enamel/dentin interface. The comprehensive cluster analysis of the spectral ranges 1752–1704 cm–1, 1718–1358 cm–1, 1269–1224 cm–1, and 1163–981 cm–1 discovered that interactions between the material and native tooth tissue were mediated by the buffer layer (fig. 6, the dotted line).
The spatial distribution of molecular and chemical phosphate, protein, Amide and ester groups (fig. 6) shows that the biomimetic layer between enamel and the dental compomer binds to the partially demineralized enamel matrix via a mediator sublayer, which may indicate an organic and mineral interaction in the analyzed region. At the same time, the data analysis (fig. 6) shows that the buffer layer between the biomimetic material and dentin is wider due to a more porous structure of dentin, as compared to enamel [1, 2]. Clusterization of IR spectra helps to see that organic and mineral components of the biomimetic buffer layer tend to permeate the light-curing adhesive agent, forming a mediator interface.
Considering our findings, one can assume that the real size of a mediator sublayer that facilitates integration between the biomimetic composite material, the restorative compomer and hard dental tissue is 3–4 μm, which is consistent with current state of scientific knowledge [27].
The data yielded by the analysis of all IR images (fig. 2–fig. 6) can be used to reliably discriminate between the functional groups of all materials present at the biomimetic system-hard native dental tissue interface and confirm the efficacy of the chosen approach to the analysis of integration processes between dental cements and next-generation biomimetic composites.
It should be noted that our findings are true for totally etched samples. However, they may be also true for the samples prepared using other techniques (self-etch or self-adhesive systems) that exploit materials similar in their chemical activity or composition to those used in our study.
CONCLUSIONS
We have demonstrated the feasibility of using multidimensional IR imaging to study the integration of next-generation biomimetic materials mimicking the mineral and organic composition of natural enamel with hard tissue of the human tooth. Using synchrotron radiation and IR maps of absorbance intensity constructed for each functional molecular group, we detected and graphically presented differences between healthy tissue, the dental cement, and the biomimetic buffer layer at their interface and determined the position and concentration of functional groups that indicate integration of the biomimetic composite with hard dental tissue. We have shown that the proposed biomimetic system consisting of nanocrystalline CSHA from a biogenic calcium source and a complex of polar amino acids found in human teeth can form a functional bond with hard tissue of human teeth. The obtained miscrospectroscopy data confirm the chemical differentiation of the materials and the presence of organic and mineral interaction at the interface between the biomimetic system and hard dental tissue.