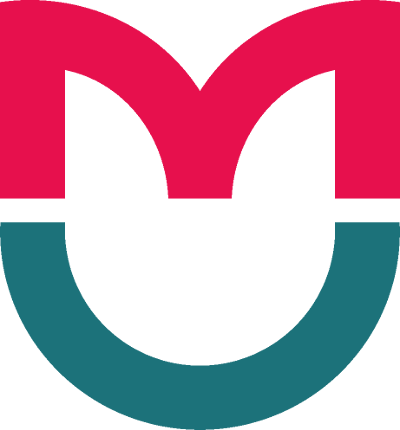
This article is an open access article distributed under the terms and conditions of the Creative Commons Attribution license (CC BY).
OPINION
Autonomous bioluminescent systems: prospects for use in the imaging of living organisms
1 Shemyakin and Ovchinnikov Institute of Bioorganic Chemistry, Moscow, Russia
2 Pirogov Russian National Research Medical University, Moscow, Russia
Correspondence should be addressed: Aleksandr S. Shcheglov
Miklukho-Маklaya, 16/10, Moscow, 117997; ur.liam@trakuj
Funding: the study was supported by the Russian Science Foundation (Grant №17-14-01169).
Acknowledgements: we thank to the Center for Precision Genome Editing and Genetic Technologies for Biomedicine (Moscow) for the genetic research methods.
Author contribution: Osipova ZM, Shcheglov AS — literature analysis, article authoring; Yampolsky IV — study planning, manuscript editing.
The use of in vivo optical imaging as a visualization method is growing in modern biomedical research [1, 2]. A non-invasive investigation of animal objects takes place during bioimaging, in which light is emitted as a result of the oxidation of a luciferin molecule (a chemical reaction catalyzed by the protein luciferase), or in response to the excitation of a fluorescent protein by light from an external source. To date the most popular optical reporter proteins are fluorescent (GFP and its homologs of different colors) as well as bioluminescent proteins from insects (FLuc) and marine organisms (RLuc, GLuc). The scope of bioluminescent and fluorescent imaging in medicine based on these proteins is very wide and includes (but is not limited to) the study of gene functions, protein-protein interactions, pathological processes and oncogenesis, drug development, etc. both in cells and tissues, as well as in lab animals real-time assays [3].
A huge variety of fluorescent proteins with different spectral properties available and a large functional toolset based on them (photoactivated, photoswitchable proteins and sensors) are the indisputable benefits of the existing fluorescence imaging technologies [4]. However, the use of fluorescent proteins requires the external source of light, resulting in the sharp decrease of sensitivity due to autofluorescence, phototoxicity and background noise. High-resolution imaging in vivo is usually accompanied by a complex invasive procedure [5]. Bioluminescent systems are free from such flaws and therefore successfully compete with fluorescent proteins. The resolution up to a single cell inside a living organism recently became available for bioluminescent imaging. Meanwhile, possible toxicity, low stability and high cost of synthetic luciferins (bioluminescent substrates), which have to be injected into the studied organism prior to every analysis, complicate bioluminescent imaging procedures.
The use of autonomously luminescent systems, for which the biosynthesis of luciferin can be reproduced by genetic engineering in the cells of the studied organism, can become an effective alternative to the existing in vivo bioimaging technologies. Unfortunately, among thousands (~103) of glowing species and around 40 (~102) different bioluminescence mechanisms, only 10 luciferins’ (glowing substrates) structures and 7 luciferases’ gene families (~101) [6, 7] are discovered to date, and the complete pathway of luciferin biosynthesis is determined only for bacterial [3] and, more recently, for fungal [8] bioluminescent systems. There is some evidence that firefly D-luciferin in insects is synthesized from p-benzoquinone and L-cysteine [9], but proteins responsible for these processes have not yet been isolated. LRE protein, regenerating D-luciferin from oxyluciferin [10, 11], cannot become an only substitute for the complete biosynthesis pathway of a luminescent substrate from common metabolites. Thus only two luminescent systems are real candidates for the development of new autonomous imaging technologies for now.
Bacterial bioluminescence: from prokaryotes to eukaryotes
Luminescent bacteria are the most widespread glowing organisms, being represented by both marine and terrestrial species. Bacterial bioluminescence is well studied: the cassette of five genes luxCDABE is responsible for the generation of light. LuxAB gene encodes a heterodimeric bacterial luciferase, luxC, luxD and luxE encode three proteins (reductase, transferase and synthase) which perform biosynthesis of the substrate (dodecanal) for bioluminescence reaction (figureA). Also, the frp gene encoding flavin reductase, responsible for the synthesis of flavin mononucleotide FMNH2, an essential component for the luminescence reaction, can also be included in the cassette [12]. Thus, bacterial bioluminescence could be completely transferred to a new organism and make it glow without the addition of luciferin from the outside. The dependence on the presence of FMNH2 and fatty aldehydes, low brightness and, most importantly, the blue color of luminescence (490 nm), which is inconvenient for in vivo deep tissue imaging, are the main disadvantages of this system.
Heterologous gene expression of an autonomous bacterial luminescent system was quickly and successfully implemented in prokaryotic cells [13], however, the large size of the operon, its multigenic organization and the toxicity of the system to the host organism (due to the cytotoxicity of dodecanal) became a fundamental difficulty for its transfer to eukaryotic cells. To solve this problem a large-scale structural rearrangement of the operon was necessary.
The first success in transferring autonomous bacterial luminescence to eukaryotes came with the transgenic luminous yeast Saccharomyces cerevisiae [14]. Efficient expression of genes was achieved by codon optimization and addition of linker regions. The choice of a thermostable luciferase of terrestrial species Photorhabdus luminescens, which remains active at 37 °C, instead of a marine one from Vibrio harveyi, was also important. Nevertheless, the glow was dim and unstable. The first successful adaptation and optimization of genes luxAB of bacterial luciferase for HEK293 cells was made after 2 years [15]. And finally, the first application of autonomous bacterial luminescence in mammalian cells for in vivo bioimaging with a sensitivity of about 20,000 cells was described in 2010 [16]. In parallel, first autonomously luminescent plants were obtained [17].
However, despite all the work done to further optimize and reduce the operon for its application in mammalian cells (for example, [18]), there is a limited number of examples of the use of bacterial bioluminescence for eukaryotic bioimaging [19–21] due to its low brightness in comparison with non-autonomous systems, for example, D-luciferin-dependent. Recently, after additional changes in the operon and the use of individual plasmids for optimized gene expression a new bacterial system “co Lux” was developed with the brightness sufficient for single-cell imaging of HEK293 cells and comparable to that of FLuc luciferase [22, 23]. Low concentration of long chain aldehyde does not lead to adverse toxic effects. The only noticeable effect was a decrease in NADPH concentration in luminous cells (due to its increased consumption during the bioluminescence reaction). The first example of autonomous bioimaging based on a fuse between LuxAB and yellow fluorescent protein YPET was also described [24].
Autonomous luminescence from fungi: an alternative to bacteria
The study of luminous fungi has a long history. For the first time, the structure of fungal luciferin (3-hydroxyhispidin) was established in 2015 [25]. In 2018 fungal luciferase and the complete cycle of luciferin biosynthesis from a widespread secondary plant metabolite caffeic acid were described, followed by the application of this system to developing autonomously luminescent yeast [8] (figureB).
The luminescence of fungi is based on the genes hisps, h3h, luz (luciferase) and cph. Hisps and h3h encode the proteins that sequentially assemble hispidin from "caffeic acid" and luciferin (3-hydroxyhyspidin), respectively. Luz encodes luciferase, and cph is responsible for the protein which converts the product of luciferin oxidation (oxyluciferin) back into caffeic acid. It was shown recently that the expression of only three genes hisps, h3h and luz in Nicotiana tabacum plants is sufficient for the creation of bright, autonomously luminescent plants [26, 27]. Unlike the bacterial luminescent system, thorough codon optimization is not necessary to obtain transgenic plants, since the genetic material is originally taken from eukaryotes and the new biosynthetic cycle fits into the metabolism of the host organism.
The first example of autonomously glowing mammalian HEK293T cells based on fungal bioluminescence genes required the use of a mixture of eight plasmids [26]. The resulting low brightness and instability of the glow indicates the necessity to further optimize the genetic constructs to achieve maximum light emission. The total length of the currently used coding sequences of the bacterial bioluminescence system is 6.2 thousand base pairs (kb), whereas for the fungal system this value is about 12.8 kb, but it can be reduced to 9.6 kb in case of utilization of alternative enzymes. Meanwhile, a significant advantage of autonomous fungal bioluminescence is the maximum of luminescence at 540 nm (yellow), which makes this system more promising for in vivo bioimaging.
CONCLUSION
The number of instruments for optical imaging in medical research is constantly increasing. In our opinion, more sensitive in vivo bioluminescent imaging in the future may compete with established fluorescence technologies. Actively studied at the moment, autonomous genetically encoded bioluminescent systems based on bacterial or fungal gene cassettes are able to be on a par with existing imaging methods.