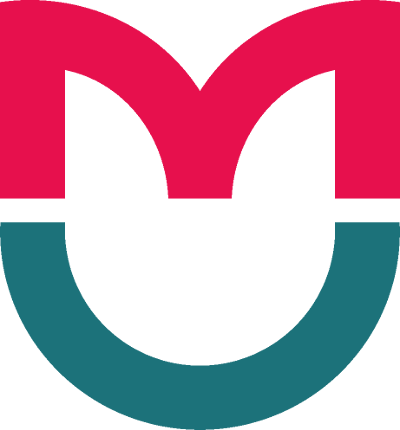
This article is an open access article distributed under the terms and conditions of the Creative Commons Attribution license (CC BY).
ORIGINAL RESEARCH
Hypoxia enhances transcytosis in intestinal enterocytes
1 National Research University Higher School of Economics, Moscow, Russia
2 P. A. Hertsen Moscow Oncology Research Center, branch of the National Medical Research Radiology Center, Moscow, Russia
3 SRC Bioclinicum, Moscow, Russia
4 Far Eastern Federal University, Vladivostok, Russia
5 Fund for Development of Innovative Scientific-Technological Center Mendeleev Valley, Moscow, Russia
Correspondence should be addressed: Diana V. Maltseva
Vavilova, 7, Moscow, 117321; moc.liamg@avestlamd
Funding: the study was supported by the Ministry of Science and Higher Education of the Russian Federation (Project ID RFMEFI61719X0056).
Acknowledgement: the authors thank the Human Proteome Core Facility (Institute of Biomedical Chemistry) for permission to use the Facility’s equipment.
Author contribution: Maltseva DV — molecular tests, analysis of their results, manuscript preparation; Shkurnikov MYu — analysis of transcriptome and sequencing data, statistical analysis; Nersisyan SA — sequencing data processing, bioinformatic analysis, functional gene analysis; Nikulin SV — cell culture, sample preparation for subsequent proteome analysis, proteomic data analysis; Kurnosov AA — sample preparation for microRNA sequencing, analysis of sequencing data; Raigorodskaya MP — real-time PCR-based analysis of gene expression, transcriptome analysis; Osipyants AI — cell culture, sample preparation for subsequent proteome and transcriptome analyses; Tonevitsky EA — supervision, data analysis, manuscript preparation.
Protecting the body against infection and creating a proper environment for commensal bacteria inhabiting the intestine are the central functions of the intestinal epithelium. [1, 2]. Bacteria and their antigens are capable of directly interacting with intestinal epithelial cells, including enterocytes [2, 3]. Such interaction can be broken down into a series of events: binding to the cell surface, endocytosis, exocytosis, and transcytosis of bacteria, their fragments or proteins [3]. Proteins, glycolipids and carbohydrates on the enterocyte surface act as receptors for bacterial pathogens [4]. There is a variety of cell lines used to model bacterial adhesion to the intestinal epithelium [5]. Of them, the most popular is the Caco-2 cell line derived from human colon adenocarcinoma cells [6–8]. In a healthy gut lumen, the hypoxic environment is maintained by the metabolic activity of commensal bacteria [5]. Low oxygen levels stabilize hypoxia-inducible factors HIF1α and HIF2α, which, in turn, affect the barrier function of the intestinal epithelium [9]. A lot of intestinal disorders are associated with hypoxia [10]. This underscores the importance of researching the interaction between the intestinal microbiota and the intestinal epithelium under low oxygen conditions.
Plant lectins are well-described proteins that are widely used to study intracellular transport and the binding of proteins to the cell surface [11, 12]. Glycosylated proteins and lipids act as receptors for plant lectins, making them a suitable model for studying bacterium-cell interactions. The aim of this work was to investigate the effect of hypoxia on transcytosis in intestinal epithelial cells.
METHODS
Immortalized Caco-2 (colon adenocarcinoma) cells (Institute of Cytology; Saint Petersburg) were cultured in a MEM medium (Gibco; USA) supplemented with 20% fetal bovine serum (FBS; Gibco; USA), 1% (v/v) solution of non-essential amino acids (Gibco; USA), 100 un/ml penicillin, and 100 μg/ml streptomycin (Gibco; USA). The cells were cultured in 6-well plates (Corning; USA) for 23 days until they differentiated into enterocyte-like cells. The medium was replaced every 2–3 days. To induce hypoxia, 300 mM CoCl2 (Sigma-Aldrich; USA) was added to the medium; the resultant mixture was incubated for 24 h. Then, the cells were washed in 1× DPBS (Gibco; USA) and lyzed for the subsequent transcriptome and proteome analysis as previously described in [13, 14].
The effects of hypoxia on the Caco-2 monolayer were evaluated using impedance spectroscopy. Prior to seeding the cells on Transwell®-96 permeable supports (Corning; USA), the wells were filled with the culture medium (50 μl in the upper compartment, 235 μl in the lower compartment) and incubated for 1 h at 37 °С in a 5% СО2 atmosphere. Then, about 5,600 cells in 50 μl medium were seeded in each insert and cultured for 23 days until they differentiated into enterocyte-like cells. The medium was replaced every 2–3 days. To induce hypoxia, CoCl2 was added to the medium as described above.
Impedance spectra were measured at room temperature in the frequency range of 40 to 20,000 Hz by means of impedance spectrometry (BioClinicum; Russia) using an STX100C96 electrode (World Precision Instruments; USA) [15]. Mean values of cell impedance properties were calculated based on the data obtained from 3 independent inserts. The membrane surface area in each well was 0.143 cm2. The data were processed using a graphical user interface for R 3.5 (R-Tools Technology; USA).
The plant lectin ricin was extracted from the seeds of the castor oil plant Ricinus communis as described in [16, 17]. Biotinylation, analysis of ricin binding to asialofetuin and cytotoxicity tests on target cells were conducted as described in [18–20].
In order to evaluate transcytosis, differentiated Caco-2 cells were co-incubated with the medium containing biotinylated ricin (1 × 10-7 М) in Transwell inserts. The protein was added into the upper compartment onto the apical cell membrane. The colonies were harvested from the lower compartment after 1 and 6 h of incubation for the subsequent immunoassay.
Lectin concentrations in the culture medium were measured using ELISA. Briefly, 100 μl of anti-ricin RA999 antibodies (10 μg/ml in 10 mM phosphate buffered saline (PBS) pH 7.4; Sigma-Aldrich; Germany) was immobilized on each well of a 96-well plate (Corning; USA) by passive adsorption. Unbound antibodies were removed by washing the wells in PBS containing 0.05% Tween-20 (PBST). To reduce non-specific binding, the wells were blocked with PBST containing 0.1% bovine serum albumin (BSA) (DiaM; Russia). Culture media were diluted 100-fold in PBST supplemented with 50 mM β-lactose (Sigma-Aldrich; Germany) and added into the wells (100 μl per well) containing the immobilized antibodies. The resultant mixtures were incubated at +37 °С in a thermal shaker. Detection of biotinylated lectin was carried out using HRP-conjugated streptavidin (Intitrogen; Germany). The antibodies were incubated in a 3,3',5,5'-tetramethylbenzidine liquid substrate (Intitrogen; Germany); the reaction was stopped with 1М НСl solution. Absorbance was measured at 450 nm wavelength using a SpectroMax i3 microplate reader (Molecular Devices; USA). Monoclonal antibodies were a gift from Professor Sveshnikov PG (Laboratory of Biotechnology, Russian Research Center for Molecular Diagnostics and Therapy; Russia).
Real-time PCR-based analysis of gene expression was performed as previously described [14]. Transcripts of the ACTB and GAPDH genes were used as references.
mRNA expression profiles were analyzed using Gene Chip Human Transcriptome Array 2.0 (TermoFisher Scientific- Affymetrix; USA). RNA isolation, quantification and quality control were performed as described in [14]. The RNA integrity number (RIN) was above 9.5 for all studied samples. cDNA was synthesized from 500 ng of isolated total RNA. All stages of sample preparation, hybridization, washing, staining, and microarray scanning were carried out as described in [21]. CEL files generated by the scanner were processed in Transcriptome Analysis Console 2.0 (TermoFisher Scientific-Affymetrix; USA). Unannotated microarray probesets were excluded from the analysis. The microarray intensity signal equal to 6.0 on the Affymetrix log scale was used as a threshold.
MicroRNA expression profiles were analyzed by means of next-generation sequencing (NGS). NGS libraries were prepared from a small RNA fraction using a CATS small RNA-seq kit (Diagenode; Belgium) following the manufacturer’s protocol. Upon PCR amplification, the libraries were purified using magnetic AMPPure beads. Final libraries concentrations were measured using a Qubit v2.0 DNA High Sensitivity DNA Kit (TermoFisher Scientific; USA). The pooled libraries were denatured, grouped on 6 Illumina HiSeq2000 V4 flow cell systems (Illumina; USA) and then sequenced. Fifty-one cycles of deep sequencing and 6 index read cycles were run. Sequencing data were exported into a FASTQ file; adapters were trimmed and poly-A-tails were removed from the reads.
Quality control of FASTQ files generated by microRNA sequencing was carried out in FastQC v0.11.9 (Babraham Bioinformatics; UK). Adapter sequences were trimmed in Cutadapt v. 2.8 [22]; reads shorter than 18 nucleotides were removed. MicroRNA expression was quantified in miRDeep2 [23]. Differential expression analysis of the microRNA sequencing data was conducted using the R environment v. 3.6.3 (R-Tools Technology; USA) and the DESeq2 library [24].
For statistical analysis, the raw data from microarrays were normalized in the oligo software package for R (R-Tools Technology; USA). Base 2 logarithm was applied to the normalized data. Differential gene and microRNA expression was analyzed using Student’s t test. False Discovery Rate (FDR) and the Benjamini-Hochberg procedure were applied for multiple testing correction.
The functional annotation of genes was performed using DAVID databases and algorithms v. 6.8 (Laboratory of Human Retrovirology and Immunoinformatics; USA). Validated microRNA: gene interactions were exported from the DIANA-TarBase database v. 8 (DIANA Lab; Greece) [25]. microRNA binding sites on 3'-non-translated regions of mRNA targets were predicted using miRWalk [26]. The search for intronic microRNA and their host genes was conducted in the miRIAD database.
Preparation of Caco-2 lysates, total protein extraction, hydrolytic cleavage and subsequent procedures necessary for proteomic analysis were carried out as described in [14]. After tryptic hydrolysis, the supernatant was collected and analyzed in a Q-Exactive HFX mass spectrometer in the positive ion mode using a nano-spray ion (nESI) source (Thermo Fisher Scientific; USA) operated at the emitter voltage of 2.1 kV and the inlet capillary temperature of 240 °C. For the analysis of differentially expressed proteins, primary spectrometry data were processed in MaxQuant 1.6 (the iBAQ algorithm) (Max- Planck-Institute of Biochemistry; Germany). Further data processing was aided by the Perseus platform and R 3.5 with the integrated RStudio 1.1 development environment (R-Tools Technology; USA). Significance of differences was evaluated using Student’s t-test.
RESULTS
Hypoxic culture conditions stimulate transcytosis across intestinal enterocytes and are associated with changed expression of genes involved in vesicle transport
Caco-2 human colon adenocarcinoma cells were cultured under hypoxia-mimicking conditions created by treating the cells with CoCl2. According to the impedance spectroscopy data, CoCl2 did not disrupt the integrity of the Сасо-2 monolayer. The transepithelial electrical resistance (TEER) value remained at 2,000 Ώ, indicating the lack of pronounced cytotoxicity and cell death.
The microarray analysis of mRNA profiles exposed that treating enterocytes with CoCl2 caused upwards of a twofold increase (FDR < 0.05) in the expression of 165 genes, among which were genes involved in cellular response to hypoxia, such as DDIT4 (2.4-fold), EGLN1 (2.7-fold), LDHA (twofold), PFKFB3 (2.1-fold), SLC2A1 (3.1-fold), SLC2A3 (3.0-fold), and VEGFA (1.4-fold). Changes in the expression profiles of these genes were confirmed by real-time PCR (p < 0.05). Besides, the functional annotation of differentially expressed genes assisted by DAVID tools revealed significant enrichment of the HIF-1 signaling pathway, which is known to mediate cellular response to hypoxia (this data is not provided in the present study). Summing up, treatment of enterocytes with CoCl2 reliably mimics low-oxygen conditions.
To assess the efficacy of transcytosis across the monolayer of enterocytes under normoxic and hypoxic conditions, the apical domain of the differentiated Сасо-2 cells grown in Transwell inserts was treated with the culture medium supplemented with biotinylated ricin. In the control wells, where the cells were cultured under normoxic conditions, the medium in the apical compartment was replaced with lectin-free medium. At 1 and 6 hours after the culture medium in the upper compartment was replaced, the medium from the lower compartment was harvested and analyzed for lectin concentrations using ELISA. Cell impedance properties were monitored for 6 h after lectin treatment; impedance spectroscopy data suggested that integrity of the monolayer was retained, which is consistent with earlier findings [27]. Six hours after lectin treatment, the TEER value increased in both control and hypoxic samples (fig. 1). This suggests formation of more intimate contacts between the cells. Thus, we can rule out the possibility of protein molecules being transported from the upper compartment containing the enterocyte monolayer into the lower compartment via paracytosis. Therefore, lectin was transported from the upper compartment to the lower by means of transcytosis.
According to ELISA, lectin undergoes transcytosis across enterocytes under both normoxic and hypoxic conditions. The amount of transcytosed lectin increased 1.8-fold (p < 0.05) after 6 h in the hypoxic environment, as compared with normoxic conditions (fig. 2).
The functional analysis of differentially expressed genes allowed us to identify 16 genes involved in the intracellular vesicular traffic (tab. 1).
Gene expression profiles of enterocytes were also analyzed by means of protein mass spectrometry. The total number of reliably detected proteins was 3,361. Levels of 237 proteins differed significantly (≥two-fold) between the samples cultured under normal and hypoxic conditions. Of all proteins with changed expression profiles (according to microarray data) that were encoded by the genes involved in the intercellular transport of vesicles, only 4 were detected in the proteome: apoB (АРОВ), SorLA-1 (SORL1), CAM-PRP (PPP3CA), and CEACAM1. A significant (6.5-fold) drop in protein expression was observed for apoB, which is consistent with the results of the transcriptome analysis.
Expression of APOB, SORL1, PPP3CA, and CEACAM1 genes in intestinal enterocytes can be regulated by miRNA
In order to identify a possible mechanism regulating the expression of genes involved in intracellular transport of vesicles, we analyzed miRNA expression profiles using NGS. Changes in transcript expression were significant (≥1.5-fold, р ≤ 0.05) for 16 miRNAs. Interestingly, 7 of them were regulatory miRNAs for APOB, SORL1, PPP3CA and CEACAM1 [25]. For these miRNAs, the direction of changes anticorrelated with the direction of changes in the expression of the corresponding mRNA targets (tab. 2).
DISCUSSION
A tremendous amount of evidence has been accrued about the mechanisms underlying the intracellular transport of vesicles by studying how ricin penetrates the cell [11]. The binding of this plant lectin to glycosylated proteins on the cell surface induces endocytosis, and ricin is then carried by the vesicles to the Golgi apparatus and endoplasmic reticulum. Thank to ricin, researchers were able to learn a lot about the apical and basolateral intracellular transport and transcytosis in polarized epithelial cells.
Ever more attention is being paid to the role of hypoxia and hypoxia-inducible signaling pathways in the physiology of the intestine and its disorders. The normal microenvironment of the intestinal epithelium is physiologically hypoxic; additional tissue hypoxia is a sign of active inflammation [10]. The integrity of the epithelial cell lining is key to the normal intestinal function [2]. But even in the absence of visible damage to the epithelial monolayer, there still could be a risk of infection due to transcytosis, e.g. the intracellular transport of vesicles containing bacteria [7, 28]. Our study demonstrates that lectin transcytosis is significantly stimulated in the intact enterocyte monolayer under hypoxic conditions. Comparative transcriptome analysis revealed changes in the expression of genes involved in the intracellular transport of vesicles in the cells cultured in a hypoxic environment. A significant drop in the expression of APOB (one of such genes) was subsequently confirmed by the proteomic analysis. Notably, the apoB protein is the key regulator of lipid and cholesterol metabolism in enterocytes [29, 30]. Found predominantly in the endoplasmic reticulum, apoB facilitates the packaging of lipids and cholesterol adsorbed from the apical lipid domain into prechylomicrons (apoB-containing particles). As these particles are transported inside membrane vesicles to the Golgi apparatus, they mature into chylomicrons and are then secreted from the basolateral enterocyte domain via exocytosis. In a healthy intestinal enterocyte, apoB-containing particles are formed and secreted continuously [29]. Perhaps, the observed significant decrease in apoB expression can activate compensatory mechanisms underlying the secretion of adsorbed and accumulated lipids and cholesterol; one of such possible mechanisms is transcytosis. Importantly, lipids and cholesterol accumulated in the cell are building blocks for cell membranes. Altogether, this might affect membrane transport in enterocytes.
In order to elucidate the mechanism regulating the observed changes, we analyzed miRNA expression profiles. Interestingly, 7 of 16 miRNAs there were found to be differentially expressed in response to hypoxia, were regulators of differentially expressed genes involved in intracellular vesicle transport. Besides, the direction of miRNA expression changes anticorrelated with changes in the expression of the corresponding mRNA-targets. Our findings suggest that enterocyte properties could be altered via a miRNA-dependent mechanism under hypoxic conditions.
CONCLUSION
Using the human intestinal enterocyte model, we demonstrated that hypoxia stimulates transcytosis. This process is accompanied by changes in the expression of genes involved in intracellular vesicle transport. We found that hypoxia is associated with a decrease in the expression of the apoB protein, the key regulator of lipid metabolism at both mRNA and protein levels. The regulatory mechanism underlying changes of cell properties might involve regulation of gene expression by microRNA. Further research into the links between lipid metabolism and transcytosis would help in designing drugs for reducing the risk of intestinal infection promoted by inflammation.