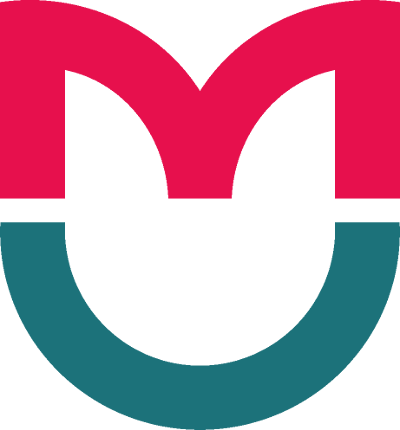
This article is an open access article distributed under the terms and conditions of the Creative Commons Attribution license (CC BY).
ORIGINAL RESEARCH
Models of mitochondrial dysfunction with inducible expression of Polg pathogenic mutant variant
1 Institute of Gene Biology, Moscow, Russia
2 Blokhin Russian Cancer Research Center, Moscow, Russia
Correspondence should be addressed: Marina V. Kubekina
Beskudnikovsky bulvar, 32, korpus 1, Moscow, 127474, Russia; moc.liamg@ymukyram
Funding: the study was supported by Russian Foundation for Basic Research, RFBR Project № 19-34-90073.
Author contribution: Kubekina MV — literature analysis, experimental research, data analysis and interpretation, oligo design, manuscript writing; Kalinina AA, Korshunova DS — experimental research; Bruter AV — literature analysis, research planning, data analysis and interpretation; Silaeva YY — literature analysis, research planning, data analysis and interpretation, scientific editing of the manuscript.
Compliance with ethical standards: the study was approved by Ethical Review board at the Institute of Gene Biology (Protocol of 05 December 2021) and carried out in strict compliance with the Directive 2010/63/EU of the European Parliament and of the Council of 22 September 2010 on the protection of animals used for scientific purposes.
Mitochondrial dysfunctions constitute a diverse group of pathological conditions, including hereditary ones; their diagnosis is complicated by variable age at manifestation and diverse symptomatics [1]. Many mitochondrial dysfunctions have been associated with mutations in mitochondrial DNA (mtDNA) [2]. Increased rates of mitochondrial mutagenesis may reflect abnormal functioning of the nuclear Polg gene responsible for mtDNA replication and repair [3]. For instance, Polg D257A genetic variant promotes accumulation of random mutations in the mitochondrial genome [4]. In fact, mitochondrial genome is generally more vulnerable than nuclear genome due to its lack of histones, authentic repair mechanisms, and increased exposure to reactive oxygen species released as by-products of aerobic respiration [5], which explains the existence of specific cellular mechanisms for the maintenance of mitochondrial genome stability. One of such mechanisms is mitophagy [6] — a type of autophagy that ensures elimination of dysfunctional mitochondria [7].
Studies on the development of mitochondrial dysfunctions and associated pathologies require appropriate models. One of the simplest in vivo modeling strategies involves creation of transgenic animals expressing a pathogenic mutant form of Polg causing disruption of mtDNA repair processes and accumulation of mitochondrial mutations. A universal model comprising genetically modified mice with inducible tissuespecific and systemic expression of the Polg D257A variant has been created to study the onset and development of mitochondrial dysfunction in various organs and tissues [8]. The design is extremely convenient, as the pathogenic mutant allele is silent unless capacitated by crossing with another transgenic line carrying activator elements, which prevents spontaneous development of the phenotype and vertical transmission of defective mitochondria.
This study aimed to evaluate the possibility of using transgenic cultures of mouse embryonic fibroblasts (MEFs) derived from Polg*Cre-CMV (Polg*B6.C-Tg(CMV-cre)1Cgn/ J mice [https://www.jax.org/strain/006054], subsequently referred to as Polg*Cre-CMV MEFs, as in vitro model of mitochondrial dysfunction caused by accumulation of mutations in mitochondrial genome promoted by expression of the mutant Polg gene. Additional goals were to evaluate the effect of systemic expression of the transgene on muscular system and various pools of T cells.
METHODS
MEF primary cultures were derived from E13.5 embryos produced by crossing Polg females with Cre-CMVs males in accordance with the published protocol [9]. All cultures used in the experiment were genotyped by PCR with primer pairs P1/P2 (STOP-cassette, 292 bp), P3/P4 (terminator, 417 bp), P5/P6 (internal control, 324 bp), and P7/P8 (CMV 100 bp); the oligonucleotide structures are listed in table.
The embryonic fibroblasts were cultured for 5 weeks (10 passages). The culture medium contained 450 mL DMEM with L-glutamine (PanEco; Russia) supplemented with 50 mL fetal calf serum (BioSera; France), penicillin-streptomycin (100X lyophilized by PanEco; Russia), and MEM non-essential amino acids (100X solution by PanEco; Russia). The mitochondrial functionalities and mitophagy levels in the studied MEF cultures were determined by serial measurements of the mitochondrial membrane potentials by fluorescence flow cytometry assay with MitoTracker Orange (Thermo Fisher Scientific; USA) and real-time PCR measurements of mitophagy marker expression levels at 7 day intervals. To measure the mitochondrial membrane potentials, the cells (105 per culture) were washed from medium in PBS (Paneco; Russia) and incubated with 100 nM MitoTracker Orange (excitation/emission = 550/580 nm) at 37 °C for 30 min, then washed from reagents, pelleted by centrifugation at 300 g for 5 min, resuspended in fresh PBS, and analyzed in a CytoFLEX flow cytometer (Beckman Coulter; USA). The results were plotted in histograms with normalization by the number of events.
Expression levels of mutated Polg in MEF cultures were determined by real time PCR assay with qPCRmix-HS (Evrogen; Russia) and primers P9/P10, using fluorescent probes P11 and P12 for the detection of wild-type and mutant variants, respectively.
During the culture period, fractions of cells (about 2 × 105 per culture) were collected weekly for RNA extraction with ExtractRNA reagent (Evrogen; Russia), reverse transcription with MMLV RT kit (Evrogen; Russia), and subsequent mitophagy gene expression assay with qPCRmix-HS SYBR kit (Evrogen; Russia) and gene-specific primers. The panel of mitophagy markers included Map1lc3a, Lamp2, Pink1, Parkin, and Nix [10]. The real-time PCR assay used genespecific primers to a reference gene Hprt (P13/P14) and the mitophagy-related genes of interest: Map1lc3a (P15/P16), Lamp2 (P17/P18), Pink1 (P19/P20), Parkin (P21/P22), and Nix (P23/P24) (see table for the structures). All manipulations with cell lysates, RNA, and cDNA were carried out in accordance with manufacturer’s protocols (Evrogen; Russia). Relative gene expression levels for mitophagy markers at different time points were determined by 2ΔCt method, with Ct (cycle threshold) corresponding to the reaction cycle number when the fluorescence crossed the threshold level; ΔCt = Ct(reference gene, Hprt) – Ct(gene of interest). Expression of a given gene of interest in control cells at a given time point was taken as a basis (= 1.0) for calculating relative expression levels in the Polg*Cre-CMV cultures [11].
We also evaluated the effects of systemic expression of the mutant Polg transgene on muscular functionalities. The experiments involved Polg*Cre-CMV transgenic line of (CBA X C57BL/6) hybrid mice developed by us previously [8] and wild-type (CBA X C57BL/6) hybrid mice purchased from the “Stolbovaya” breeding facilities. Only female mice, aged 3 months, were used in the experiments. The animals, weighting 19.9 ± 2.2 g (Polg*Cre-CMV) and 22.7 ± 2.5 g (wild-type), were housed at the Institute of Gene Biology animal facilities with ad libitum access to food and water, 12/12 light cycle, 23 ± 1 °C air temperature, and 42 ± 5% humidity. Each group of the study (5 animals) was subject to “grip strength” and “wire hang” tests. The wire hang test was carried out as described in [12] with modifications (the total time for 10 attempts was limited to 300 s). The grip strength test was carried out as described in [13] with modifications (a grid was used instead of a bar). All animals were sacrificed by cervical dislocation; the spleens and the thymuses were dissected for subsequent examination.
Functional state of the immune system was assessed indirectly by measuring gene expression levels for T cell receptor (TCR) subunit α and CD3 coreceptor complex subunits δ and ε in total splenocytes of Polg*Cre-CMV mice and matched wildtype controls. We also analyzed representation of CD4/CD8 double-negative, double-positive, and single-positive cell populations of the thymus along with its absolute cellularity. The mice were sacrificed by cervical dislocation; the abdominal wall was cut open with scissors; the spleen and the thymus were carefully dissected and thoroughly homogenized. The splenic cells were lyzed for RNA extraction and reverse transcription PCR assay using the reagents and protocols listed in the previous paragraphs. The real-time PCR assay used genespecific primers to the reference gene Hprt (P13/P14) and lymphocyte markers Cd3δ (/P26), Cd3ε (P27/P28), and Tcr-α (P29/P30) (see table for the structures). Relative expression levels were calculated by 2ΔCt method described in the previous paragraphs. Single-cell suspensions of thymus cells were analyzed by flow cytometry as described elsewhere [14]. The thymus cells were counted in a Goryaev chamber.
Statistical processing of the data was carried out in Excel (Microsoft; USA) and Statistica (Statsoft; Russia) program packages. The normality of distributions was tested with Shapiro–Wilk test. The samples were characterized using nonparametric one-way two-sample analysis and Mann– Whitney U-test for between-the group comparisons. The differences were considered significant at p ≤ 0.05.
RESULTS
All obtained MEF cultures were genotyped; the results are shown in fig. 1A, B. In Polg*Cre-CMV double transgenes, the STOP cassette is excised due to systemic activation of Cre recombinase, leaving the embryos STOP cassette-negative and internal control-, terminator-, and CMV-positive. The individual MEF cultures derived from different embryos were distributed into groups based on genotype: eight Polg*Cre-CMV cultures constituted the main group; the rest (three Polg, three CreCMV, and four wild-type) were assigned to the control group.
Expression levels of the Polg mutant variant were measured in all cultures (fig. 1C). The mutant variant was strongly expressed in cultures genotyped as Polg*Cre-CMV (main group) and none of the controls. The observed differences were significant (p ≤ 0.05).
Dynamics of the mitochondrial membrane potential in MEF cultures are shown in fig. 1D. The mitochondrial membrane potential of Polg*Cre-CMV MEFs was significantly reduced starting from week 3 of culturing compared with the controls (p ≤ 0.05).
Comparison of expression profiles for the panel of mitophagy markers revealed significant between-the-group differences (fig. 2A–E):
- Expression of Pink1/Parkin genes, the well-known markers of mitophagy, gradually increased over time (fig. 2A, B), consistently with the presumed increase in the number of dysfunctional mitochondria [15].
- Accumulation of random mutations in the mitochondrial genome promotes a decrease in oxidative phosphorylation, which mimics hypoxia, with a concomitant increase in Nix expression [16]. The sharp increase in Nix expression recorded during week 3 of culturing was probably induced by critically accumulated intermediates of the disrupted energy-dependent processes (fig. 2C). In mitophagy, the Map1lc3a gene expression product binds Nix protein anchored in the outer mitochondrial membrane, thus tethering it to autophagosome. This protein is also known to initiate the autophagy [17], which explains the sharp positive dynamics of Map1lc3a expression during week 2 and its subsequent correlation with Nix expression levels (fig. 2D). This does not explain, however, the observed decrease of Nix and Map1lc3a expression in transgenic MEFs during weeks 4 and 5 of culturing. Apparently, the decrease reflects the stalled elimination of the mitochondrial ballast (progressively dysfunctional) due to physiological stress and the loss of viability.
- Lamp2 protein is a structural component of the lysosomal membrane [18]. Expression of the corresponding Lamp2 gene in Polg*Cre-CMV cultures exceeded its expression in control cultures 2-fold on the first week of culturing and stayed elevated, apparently reflecting the increased number of lysosomes required for efficient autophagy (fig. 2E).
To study the impact of mitochondrial dysfunction on muscular functionalities in Polg*Cre-CMV transgenic animals, we tested muscular endurance and grip strength in Polg*Cre-CMV transgenic mice compared with wild-type controls. The results of wire hang test are shown in fig. 3A. Wild-type mice held on the wire throughout the experiment with a maximum loss of 4 points, while Polg*Cre-CMV mice performed significantly worse: the animals quitted the task in 199 s on average. In grip strength tests, Polg*Cre-CMV mice also performed significantly worse than wild-type controls (p ≤ 0.05) (fig. 3B).
To assess the influence of activated transgene on peripheral pools of T cells, we studied expression of genes encoding ε and δ subunits of CD3 complex and α chain of TCR in total splenocytes of Polg*Cre-CMV animals compared with wild-type controls. The levels of Cd3ε, Cd3δ, and Tcr-α expression in lymphocytes of Polg*Cre-CMV mice were significantly reduced compared with wild-type controls (p ≤ 0.05) (fig. 4). The fluorescence flow cytometry of single-cell suspensions of viable thymus cells revealed no shifts in CD4/CD8 doublenegative, double-positive, and single-positive subpopulation ratio (fig. 5A, B); however, the absolute cellularity of the thymus in Polg*Cre-CMV mice was significantly lower (p ≤ 0.05) (fig. 5C).
DISCUSSION
Development of mitochondrial dysfunction through accumulation of mutations in the mitochondrial genome affects almost all organs and tissues. The alterations caused by mitochondrial mutations begin in embryogenesis. Our experiments indicate that primary cultures of embryonic fibroblasts with Polg*Cre-CMV genotype develop mitochondrial dysfunction in the course of 3 weeks. This effect entirely results from expression of the pathogenic mutant variant, given its absence in control cultures. Thus, embryonic fibroblast cultures derived from Polg*Cre-CMV transgenic mice represent a convenient in vitro model for studying mitochondrial dysfunction caused by accumulation of harmful mutations in the mitochondrial genome.
High mitochondrial efficiency is essential for normal functioning of tissues and organ systems, notably the immune system and the muscles [19, 20]. Our experiments demonstrate a considerable negative impact of pathogenic mutations in Polg on the functional state of muscular apparatus, revealed by decreased muscle endurance and grip strength in animals with systemic expression of the Polg*Cre-CMV transgene compared with wild-type controls. The transgene also had a negative effect on the immune system: decreased expression levels of δ and ε subunits of CD3 complex and α chain of T cell receptor in splenocytes indirectly indicate reduced functionality of peripheral T cells in Polg*Cre-CMV transgenic animals. Examination of the thymus in transgene-positive animals revealed accelerated involution of this organ with preservation of its main function. These findings are consistent with the reported association of mitochondrial mutagenesis with progeroid phenotypes [4]. Overall, the results justify the use of Polg*Cre-CMV transgenic animals with inducible expression of mutated Polg as a relevant model for studying mitochondrial dysfunction and its consequences.
CONCLUSIONS
Expression of the mutated Polg gene promotes an increase in mitophagy and a concomitant decrease in the mitochondrial membrane potential in cultured mouse embryonic fibroblasts, as revealed by a series of experiments involving Polg*CreCMV transgenic animals. The wire hang and grip strength functional tests revealed significantly reduced muscular endurance in Polg*Cre-CMV mice compared with wild-type controls. Expression of the mutant Polg gene also affects immune functionalities, as indicated by reduced TCR/CD3 gene expression in peripheral lymphocytes of Polg*Cre-CMV mice. Systemic expression of the transgene affects cellularity of the thymus, albeit not its CD4/CD8 subpopulation ratio. The obtained results are convincing; however, it would be interesting to study effects of the mutant Polg gene in other organs and systems. The dedicated in vitro and in vivo models are needed to improve our understanding of mitochondrial dysfunctions and their therapy.